
- •Analysis and Application of Analog Electronic Circuits to Biomedical Instrumentation
- •Dedication
- •Preface
- •Reader Background
- •Rationale
- •Description of the Chapters
- •Features
- •The Author
- •Table of Contents
- •1.1 Introduction
- •1.2 Sources of Endogenous Bioelectric Signals
- •1.3 Nerve Action Potentials
- •1.4 Muscle Action Potentials
- •1.4.1 Introduction
- •1.4.2 The Origin of EMGs
- •1.5 The Electrocardiogram
- •1.5.1 Introduction
- •1.6 Other Biopotentials
- •1.6.1 Introduction
- •1.6.2 EEGs
- •1.6.3 Other Body Surface Potentials
- •1.7 Discussion
- •1.8 Electrical Properties of Bioelectrodes
- •1.9 Exogenous Bioelectric Signals
- •1.10 Chapter Summary
- •2.1 Introduction
- •2.2.1 Introduction
- •2.2.4 Schottky Diodes
- •2.3.1 Introduction
- •2.4.1 Introduction
- •2.5.1 Introduction
- •2.5.5 Broadbanding Strategies
- •2.6 Photons, Photodiodes, Photoconductors, LEDs, and Laser Diodes
- •2.6.1 Introduction
- •2.6.2 PIN Photodiodes
- •2.6.3 Avalanche Photodiodes
- •2.6.4 Signal Conditioning Circuits for Photodiodes
- •2.6.5 Photoconductors
- •2.6.6 LEDs
- •2.6.7 Laser Diodes
- •2.7 Chapter Summary
- •Home Problems
- •3.1 Introduction
- •3.2 DA Circuit Architecture
- •3.4 CM and DM Gain of Simple DA Stages at High Frequencies
- •3.4.1 Introduction
- •3.5 Input Resistance of Simple Transistor DAs
- •3.7 How Op Amps Can Be Used To Make DAs for Medical Applications
- •3.7.1 Introduction
- •3.8 Chapter Summary
- •Home Problems
- •4.1 Introduction
- •4.3 Some Effects of Negative Voltage Feedback
- •4.3.1 Reduction of Output Resistance
- •4.3.2 Reduction of Total Harmonic Distortion
- •4.3.4 Decrease in Gain Sensitivity
- •4.4 Effects of Negative Current Feedback
- •4.5 Positive Voltage Feedback
- •4.5.1 Introduction
- •4.6 Chapter Summary
- •Home Problems
- •5.1 Introduction
- •5.2.1 Introduction
- •5.2.2 Bode Plots
- •5.5.1 Introduction
- •5.5.3 The Wien Bridge Oscillator
- •5.6 Chapter Summary
- •Home Problems
- •6.1 Ideal Op Amps
- •6.1.1 Introduction
- •6.1.2 Properties of Ideal OP Amps
- •6.1.3 Some Examples of OP Amp Circuits Analyzed Using IOAs
- •6.2 Practical Op Amps
- •6.2.1 Introduction
- •6.2.2 Functional Categories of Real Op Amps
- •6.3.1 The GBWP of an Inverting Summer
- •6.4.3 Limitations of CFOAs
- •6.5 Voltage Comparators
- •6.5.1 Introduction
- •6.5.2. Applications of Voltage Comparators
- •6.5.3 Discussion
- •6.6 Some Applications of Op Amps in Biomedicine
- •6.6.1 Introduction
- •6.6.2 Analog Integrators and Differentiators
- •6.7 Chapter Summary
- •Home Problems
- •7.1 Introduction
- •7.2 Types of Analog Active Filters
- •7.2.1 Introduction
- •7.2.3 Biquad Active Filters
- •7.2.4 Generalized Impedance Converter AFs
- •7.3 Electronically Tunable AFs
- •7.3.1 Introduction
- •7.3.3 Use of Digitally Controlled Potentiometers To Tune a Sallen and Key LPF
- •7.5 Chapter Summary
- •7.5.1 Active Filters
- •7.5.2 Choice of AF Components
- •Home Problems
- •8.1 Introduction
- •8.2 Instrumentation Amps
- •8.3 Medical Isolation Amps
- •8.3.1 Introduction
- •8.3.3 A Prototype Magnetic IsoA
- •8.4.1 Introduction
- •8.6 Chapter Summary
- •9.1 Introduction
- •9.2 Descriptors of Random Noise in Biomedical Measurement Systems
- •9.2.1 Introduction
- •9.2.2 The Probability Density Function
- •9.2.3 The Power Density Spectrum
- •9.2.4 Sources of Random Noise in Signal Conditioning Systems
- •9.2.4.1 Noise from Resistors
- •9.2.4.3 Noise in JFETs
- •9.2.4.4 Noise in BJTs
- •9.3 Propagation of Noise through LTI Filters
- •9.4.2 Spot Noise Factor and Figure
- •9.5.1 Introduction
- •9.6.1 Introduction
- •9.7 Effect of Feedback on Noise
- •9.7.1 Introduction
- •9.8.1 Introduction
- •9.8.2 Calculation of the Minimum Resolvable AC Input Voltage to a Noisy Op Amp
- •9.8.5.1 Introduction
- •9.8.5.2 Bridge Sensitivity Calculations
- •9.8.7.1 Introduction
- •9.8.7.2 Analysis of SNR Improvement by Averaging
- •9.8.7.3 Discussion
- •9.10.1 Introduction
- •9.11 Chapter Summary
- •Home Problems
- •10.1 Introduction
- •10.2 Aliasing and the Sampling Theorem
- •10.2.1 Introduction
- •10.2.2 The Sampling Theorem
- •10.3 Digital-to-Analog Converters (DACs)
- •10.3.1 Introduction
- •10.3.2 DAC Designs
- •10.3.3 Static and Dynamic Characteristics of DACs
- •10.4 Hold Circuits
- •10.5 Analog-to-Digital Converters (ADCs)
- •10.5.1 Introduction
- •10.5.2 The Tracking (Servo) ADC
- •10.5.3 The Successive Approximation ADC
- •10.5.4 Integrating Converters
- •10.5.5 Flash Converters
- •10.6 Quantization Noise
- •10.7 Chapter Summary
- •Home Problems
- •11.1 Introduction
- •11.2 Modulation of a Sinusoidal Carrier Viewed in the Frequency Domain
- •11.3 Implementation of AM
- •11.3.1 Introduction
- •11.3.2 Some Amplitude Modulation Circuits
- •11.4 Generation of Phase and Frequency Modulation
- •11.4.1 Introduction
- •11.4.3 Integral Pulse Frequency Modulation as a Means of Frequency Modulation
- •11.5 Demodulation of Modulated Sinusoidal Carriers
- •11.5.1 Introduction
- •11.5.2 Detection of AM
- •11.5.3 Detection of FM Signals
- •11.5.4 Demodulation of DSBSCM Signals
- •11.6 Modulation and Demodulation of Digital Carriers
- •11.6.1 Introduction
- •11.6.2 Delta Modulation
- •11.7 Chapter Summary
- •Home Problems
- •12.1 Introduction
- •12.2.1 Introduction
- •12.2.2 The Analog Multiplier/LPF PSR
- •12.2.3 The Switched Op Amp PSR
- •12.2.4 The Chopper PSR
- •12.2.5 The Balanced Diode Bridge PSR
- •12.3 Phase Detectors
- •12.3.1 Introduction
- •12.3.2 The Analog Multiplier Phase Detector
- •12.3.3 Digital Phase Detectors
- •12.4 Voltage and Current-Controlled Oscillators
- •12.4.1 Introduction
- •12.4.2 An Analog VCO
- •12.4.3 Switched Integrating Capacitor VCOs
- •12.4.6 Summary
- •12.5 Phase-Locked Loops
- •12.5.1 Introduction
- •12.5.2 PLL Components
- •12.5.3 PLL Applications in Biomedicine
- •12.5.4 Discussion
- •12.6 True RMS Converters
- •12.6.1 Introduction
- •12.6.2 True RMS Circuits
- •12.7 IC Thermometers
- •12.7.1 Introduction
- •12.7.2 IC Temperature Transducers
- •12.8 Instrumentation Systems
- •12.8.1 Introduction
- •12.8.5 Respiratory Acoustic Impedance Measurement System
- •12.9 Chapter Summary
- •References

Sources and Properties of Biomedical Signals |
|
|
|
17 |
|
|
Rtip |
Eel |
|
Rpe |
|
|
Rtc |
+ Rse |
|
|
|
|
|
|
(E) |
||
|
|
|
|
Cpe |
|
+ |
|
|
|
|
|
|
|
|
|
|
|
Etip |
|
|
|
|
+ |
(Inside cell) |
|
|
|
|
|
|
|
|
|
|
|
Cc |
Gc |
∆Ctipk |
|
|
Ve |
|
|
|
|||
Im |
|
|
|
|
|
|
|
|
|
Rpe |
− |
|
|
Eel |
Rse |
|
|
|
|
|
|
||
|
|
|
|
Cpe |
(R) |
|
(Outside cell) |
|
+ |
|
|
|
|
|
|
A
R
(E)
+
Vbio |
C |
Ve |
−
(R)
B
FIGURE 1.7
(A) Equivalent circuit of an intracellular glass microelectrode in a cell, including the equivalent circuits of the AgΩAgCl electrodes. Note the dc half-cell potentials of the electrodes and at the microelectrode’s tip. The tip of the microelectrode is modeled by a lumped-parameter, nonuniform, R–C transmission line. (B) For practical purposes, the ac equivalent circuit of the glass micropipette electrode is generally reduced to a simple R–C low-pass filter.
Typical values of circuit parameters are Rμ 2 ∞ 108 Ω, Cμ 1 ∞ 10−12 F. Thus fb 796 Hz. This is too low a break frequency to reproduce a nerve action potential faithfully, which requires at least a dc to 3-kHz bandwidth. To obtain the desired bandwidth, capacitance neutralization is used (capacitance neutralization is described in Section 4.5.2 of Chapter 4). As shown in Section 9.8.4 in Chapter 9, capacitance neutralization adds excess noise to the amplifier output.
1.9Exogenous Bioelectric Signals
The preceding sections have shown that endogenous bioelectric signals are invariably small, ranging from single microvolts to over 100 mV. Their bandwidths range from dc to perhaps 10 kHz at the most. Signals such as ECG
© 2004 by CRC Press LLC
18 |
Analysis and Application of Analog Electronic Circuits |
and EEG require approximately 0.1 to 100 Hz. Exogenous signals on the other hand, can involve modalities such as ultrasound, which can use frequencies from hundreds of kilohertz to tens of megahertz, depending on the application. Ultrasound can be continuous wave (CW) sinusoidal, sinusoidal pulses, or wavelets. The purpose here is not to discuss the details of how ultrasound is generated, received, or processed, but rather to comment on the frequencies and signal levels of the received ultrasound.
Reflected ultrasound is picked up by a piezoelectric transducer or transducer array that converts the mechanical sound pressure waves at the skin surface to electrical currents or voltages. Concern for damaging tissues with the transmitted ultrasound intensity (cells destroyed by heating or cavitation) means that input sound intensity must be kept low enough to be safe for living tissues, organs, fetuses, etc., yet high enough to give a good output signal SNR from the reflected sound energy impinging on the receiving transducer. Many ultrasound transducers are used at ultrasound frequencies below their mechanical resonance.
In this region of operation, the transducer has an equivalent circuit described by Figure 1.8. Cx is the capacitance of the transducer, which of course depends on thickness, dielectric constant of its material, and area of the metal film electrodes; Cx is generally on the order of hundreds of picofarads. Gx is the leakage conductance of the piezomaterial and also depends on the material and its dimensions. Expect Gx on the order of 10−13 S. The coaxial cable connecting the transducer to the charge amplifier has some shunt capacitance, Cc, which depends on cable length and insulating material; it will be on the order of approximately 30 pF/m. Similarly, the cable has some leakage conductance, Gc, which again is length and material dependent; Gc will be about 10−11 S. Finally, the input conductance and capacitance of the electrometer op amp are about Gi = 10−14 S and Ci = 3 pF.
The circuit of Figure 1.8 is a charge amplifier, which effectively replaces
(Cx + Cc + Ci) and (Gx + Gc + Gi) with CF and GF in parallel with the transducer’s Norton current source. Thus the low-frequency behavior of the sys-
tem is not set by the poorly defined (Cx + Cc + Ci) and (Gx + Gc + Gi) but rather by the designer-specified components GF and CF. (Analysis of the circuit is carried out in detail in Section 6.6.3 of Chapter 6.) Note that the Norton current source, ix, is proportional to the rate of change of the ultrasound pressure waves impingent on the bottom of the transducer. The constant d has the dimensions of coulombs/newton. The charge displaced inside
the transducer is given by q = d F. The Norton current is simply ix ∫ q· = d F· =
·
d PA. If the op amp is assumed to be ideal, then the summing junction is at 0 V and, by Ohm’s law, the op amp’s output voltage is
V = |
dAsP(s) |
(1.2) |
|
|
|||
o |
sCF |
+ GF |
|
|
|
© 2004 by CRC Press LLC

Sources and Properties of Biomedical Signals |
19 |
Voc
(Metal film electrodes)
Piezo-transducer
Ultrasound gel
Skin
Tissue
Pi
|
|
|
GF |
|
|
|
CF |
|
|
|
(0) |
• |
Cx |
Cc + Ci |
Vo |
ix = Pd |
|
Gx |
OA |
|
|
|
Gc + Gi |
(Cable)
FIGURE 1.8
Top: Cross-sectional schematic of a piezoelectric transducer on the skin surface. The gel is used for acoustic impedance matching to improve acoustic signal capture efficiency. Pi is the sound pressure of the signal being sensed. Bottom: Equivalent circuit of the piezosensor and a charge amplifier. See text for analysis.
Written as a transfer function, this is |
|
|
|
|||||||
|
|
|
Vo |
(s) = |
|
dAsRF |
(1.3) |
|||
|
|
|
P |
sC |
R + 1 |
|
||||
|
|
|
|
|
|
|
|
F F |
|
|
At mid-frequencies, the gain is: |
|
|
|
|
|
|
|
|||
|
Vo |
(s) |
= |
dA |
volt pascal |
(1.4) |
||||
|
|
|
||||||||
|
P |
|
CF |
|
|
|
The area A is in m2. The parameter d varies considerably between piezomaterials and also depends on the direction of cut in natural crystals such as quartz, Rochelle salt, and ammonium dihydrogen phosphate. For example, d for X-cut quartz crystals is 2.25 ∞ 10−12 Cb/N and d for barium titanate is approximately 160 ∞ 10−12 Cb/N.
Now consider the mid-frequency output of the charge amplifier when using a lead–zirconate–titanate (LZT) transducer having d = 140 ∞ 10−12 Cb/N, given a sound pressure of 1 dyne/cm2 = 0.1 Pa; A = 1 cm2 = 10−4 m2; and CF = 100 pF.
© 2004 by CRC Press LLC

20 |
|
Analysis and Application of Analog Electronic Circuits |
||||
V = |
PAd |
= |
0.1 ∞ 10−4 ∞ 140 ∞ 10−12 |
= 14 μV |
(1.5) |
|
C |
|
100 ∞ 10−12 |
||||
o |
F |
|
|
|
||
|
|
|
|
|
|
Thus, received voltage levels in piezoelectric ultrasonic systems are very low. Considerable low-noise amplification is required and band-pass filtering is necessary to improve signal-to-noise ratio. Not included in the preceding analysis is the high-frequency response of the charge amplifier, which is certainly important when conditioning low-level ultrasonic signals in the range of megahertz. (See Section 6.6.3 in Chapter 6 for high-frequency analysis of the charge amplifier.)
1.10 Chapter Summary
This chapter has stressed that endogenous biomedical signals, whether electrical such as the ECG or EEG, or physical quantities such as blood pressure or temperature, vary relatively slowly; their signal bandwidths are generally from dc to several hundred Hertz and, in the case of nerve spikes or EMGs, may require up to 2 to 4 kHz at the high end. All bioelectric signals are noisy — that is, they are recorded in the company of broadband noise arising from nearby physiological sources; in many cases (e.g., EEG, ERG, EOG, and ECoG), they are in the microvolt range and must compete with amplifier noise. In the case of a skin-surface EMG, the recorded signals are the spa- tio–temporal summation of many thousand individual sources underlying the electrodes, i.e., many muscle fibers asynchronously generating action potentials as they contract to do mechanical work. In the case of the scalprecorded EEG, many millions of cortical neurons signal by spiking or by slow depolarization as the brain works, generating a spatio–temporally summed potential between pairs of scalp electrodes.
On the other hand, the electrical activity from the heart is spatially localized. The synchronous spread of depolarization of cardiac muscle during the cardiac cycle generates a relatively strong signal on the skin surface, in spite of the large volume conductor volume through which the ECG electric field must spread. Figure 1.9 illustrates the approximate extreme ranges of peak signal amplitudes and the approximate range of frequencies required to condition EOG, EEG, ECG, and EMG signals. Note that the waveforms that contain spikes or sharp peak transients (ECG and EMG) require a higher bandwidth to characterize.
Exogenous biomedical instrumentation signals, such as diagnostic and Doppler ultrasound and signals from MRI systems, were shown to require bandwidths into the tens of megahertz and higher. Amplifiers required for
conditioning such exogenous signals must have high gain bandwidth products (fT), and high slew rates (η), as well as low noise.
© 2004 by CRC Press LLC
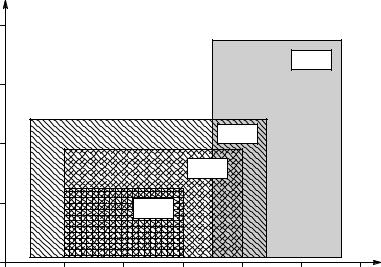
Sources and Properties of Biomedical Signals |
21 |
Voltage |
|
|
|
|
|
|
range |
|
|
|
|
|
|
10−1 |
|
|
|
|
|
|
|
|
|
|
|
EMG |
|
10−2 |
|
|
|
|
|
|
10−3 |
|
|
|
ECG |
|
|
|
|
|
|
|
|
|
|
|
|
|
EEG |
|
|
10−4 |
|
|
EOG |
|
|
|
|
|
|
|
|
|
|
|
|
|
|
|
|
f (Hz) |
10−5 |
|
|
|
|
|
|
10−2 |
0.1 |
1.0 |
10 |
102 |
103 |
104 |
FIGURE 1.9
Approximate RMS spectra of four classes of bioelectric signals. Peak expected RMS signal is plotted vs. extreme range of frequencies characterizing the signal.
© 2004 by CRC Press LLC