
- •VOLUME 1
- •CONTRIBUTOR LIST
- •PREFACE
- •LIST OF ARTICLES
- •ABBREVIATIONS AND ACRONYMS
- •CONVERSION FACTORS AND UNIT SYMBOLS
- •ABLATION.
- •ABSORBABLE BIOMATERIALS.
- •ACRYLIC BONE CEMENT.
- •ACTINOTHERAPY.
- •ADOPTIVE IMMUNOTHERAPY.
- •AFFINITY CHROMATOGRAPHY.
- •ALLOYS, SHAPE MEMORY
- •AMBULATORY MONITORING
- •ANALYTICAL METHODS, AUTOMATED
- •ANALYZER, OXYGEN.
- •ANESTHESIA MACHINES
- •ANESTHESIA MONITORING.
- •ANESTHESIA, COMPUTERS IN
- •ANGER CAMERA
- •ANGIOPLASTY.
- •ANORECTAL MANOMETRY
- •ANTIBODIES, MONOCLONAL.
- •APNEA DETECTION.
- •ARRHYTHMIA, TREATMENT.
- •ARRHYTHMIA ANALYSIS, AUTOMATED
- •ARTERIAL TONOMETRY.
- •ARTIFICIAL BLOOD.
- •ARTIFICIAL HEART.
- •ARTIFICIAL HEART VALVE.
- •ARTIFICIAL HIP JOINTS.
- •ARTIFICIAL LARYNX.
- •ARTIFICIAL PANCREAS.
- •ARTERIES, ELASTIC PROPERTIES OF
- •ASSISTIVE DEVICES FOR THE DISABLED.
- •ATOMIC ABSORPTION SPECTROMETRY.
- •AUDIOMETRY
- •BACTERIAL DETECTION SYSTEMS.
- •BALLOON PUMP.
- •BANKED BLOOD.
- •BAROTRAUMA.
- •BARRIER CONTRACEPTIVE DEVICES.
- •BIOCERAMICS.
- •BIOCOMPATIBILITY OF MATERIALS
- •BIOELECTRODES
- •BIOFEEDBACK
- •BIOHEAT TRANSFER
- •BIOIMPEDANCE IN CARDIOVASCULAR MEDICINE
- •BIOINFORMATICS
- •BIOLOGIC THERAPY.
- •BIOMAGNETISM
- •BIOMATERIALS, ABSORBABLE
- •BIOMATERIALS: AN OVERVIEW
- •BIOMATERIALS: BIOCERAMICS
- •BIOMATERIALS: CARBON
- •BIOMATERIALS CORROSION AND WEAR OF
- •BIOMATERIALS FOR DENTISTRY
- •BIOMATERIALS, POLYMERS
- •BIOMATERIALS, SURFACE PROPERTIES OF
- •BIOMATERIALS, TESTING AND STRUCTURAL PROPERTIES OF
- •BIOMATERIALS: TISSUE-ENGINEERING AND SCAFFOLDS
- •BIOMECHANICS OF EXERCISE FITNESS
- •BIOMECHANICS OF JOINTS.
- •BIOMECHANICS OF SCOLIOSIS.
- •BIOMECHANICS OF SKIN.
- •BIOMECHANICS OF THE HUMAN SPINE.
- •BIOMECHANICS OF TOOTH AND JAW.
- •BIOMEDICAL ENGINEERING EDUCATION
- •BIOSURFACE ENGINEERING
- •BIOSENSORS.
- •BIOTELEMETRY
- •BIRTH CONTROL.
- •BLEEDING, GASTROINTESTINAL.
- •BLADDER DYSFUNCTION, NEUROSTIMULATION OF
- •BLIND AND VISUALLY IMPAIRED, ASSISTIVE TECHNOLOGY FOR
- •BLOOD BANKING.
- •BLOOD CELL COUNTERS.
- •BLOOD COLLECTION AND PROCESSING
- •BLOOD FLOW.
- •BLOOD GAS MEASUREMENTS
- •BLOOD PRESSURE MEASUREMENT
- •BLOOD PRESSURE, AUTOMATIC CONTROL OF
- •BLOOD RHEOLOGY
- •BLOOD, ARTIFICIAL
- •BONDING, ENAMEL.
- •BONE AND TEETH, PROPERTIES OF
- •BONE CEMENT, ACRYLIC
- •BONE DENSITY MEASUREMENT
- •BORON NEUTRON CAPTURE THERAPY
- •BRACHYTHERAPY, HIGH DOSAGE RATE
- •BRACHYTHERAPY, INTRAVASCULAR
- •BRAIN ELECTRICAL ACTIVITY.
- •BURN WOUND COVERINGS.
- •BYPASS, CORONARY.
- •BYPASS, CARDIOPULMONARY.
540BONE CEMENT, ACRYLIC
169.McLeod KJ, Rubin CT. The effect of low frequency electrical fields on osteoporosis. J Bone Joint Surg 1992;74-A:920–929.
170.Skerry TM, Pead MJ, Lanyon LE. Modulation of bone loss during disuse by pulsed electromagnetic fields. J Orthop Res 1991;9:600–608.
171.Granhed H, Jonson R, Hansson T. The loads on the lumbar spine during extreme weight lifting., Spine 1987;12(2): 146–149.
Reference List
Gilmore RS, Katz JL. Elastic properties of apatites. J Mat Sci 1982;17:1131–1141.
Katz JL, Ukraincik K. On the anisotropic elastic properties of hydroxyapatite. J Biomech 1971;4:221–227.
Lakes RS, Katz JL. Viscoelastic properties of bone. In Hastings G, Ducheyne P, editors. Natural and Living Biomaterials. Washington, (DC): CRC Press; 1984.
Ortmann R, Perkins JP. Stimulation of adenosine 30:50 monophospate formation by prostaglandins in human astrocytoma cells. J Biol Chem 1977;252:6019–6025.
See also BIOMATERIALS FOR DENTISTRY; BONE CEMENT, ACRYLIC; TOOTH AND JAW, BIOMECHANICS OF.
BONE CEMENT, ACRYLIC
CHAODI LI
YAN ZHOU
University of Notre Dame
Notre Dame, Indiana
INTRODUCTION
Many years of intensive research by Rohm led to the development of poly(methyl methacrylate) (PMMA), the basis of bone cement, in 1934 (1,2). This polymer was reportedly first used to close cranial defects in monkeys in a medical application in the late 1930s (2). The use of acrylic bone cement in orthopedic surgery was first advocated in 1951 by Kiaer and Jansen. It was applied as pure anchoring material by fixing acrylic glass caps on the femoral head after removing the cartilage (2–4). At that time, the femoral components in total hip replacements were still implanted by simply press-fitting the prosthesis tightly into the prepared intramedullary (marrow) canal of the femur. Patients were confined to bed for a relatively long period of time after surgery and often experienced pain later from loosening of the implant. In 1958, Sir John Charnley first applied the self-curing bone cement for the fixation of artificial joints (4). In this surgery, the cement filled the free space between the prosthesis and the bone
(2). Bone cement served as a mechanical interlock between the metallic prosthesis and the bone and it has been found to be an appropriate material to transfer the load consistently (5). In 1969, bone cement was approved for general use within the United States and since then the number of total hip and knee replacements has increased dramatically (3). Currently, it is the only material used for anchoring cemented arthroplasties to the contiguous bones (6). More than one-half million hip replacement surgeries are performed every year worldwide and 70% of the surgeries are performed using bone cements (7–10).
Bone cement is also extensively used in the fixation of pathological fractures, spinal surgery, and neurosurgery (11). Every year, osteoporosis results in > 310,000 fractures in the United Kingdom alone (12). Vertebral compression fractures occur in 20% of people over the age of 70 years and in 16% of postmenopausal women (12). Although traditional conservative techniques, such as bed rest and the prescription of analgesics may be successful in a proportion of cases, a significant number of sufferers remain in long-term pain. Vertebroplasty is now being used extensively for vertebral compression fracture treatments (12,13). This technique entails the percutaneous injection of bone cement into the fractured vertebra in attempts to stabilize the fracture and reduce pain. Studies have reported excellent pain relief and improved function in most patients (12,13). Bone cements have also been applied as an adjunct to internal fixation for treating fractures. Bone cement fills voids in bone, thereby reducing the need for bone grafts, and may improve the holding strength around the devices in osteoporotic bone. Recently, the technique of PMMA bone cement injection into the osteoporotic proximal femur was proposed (14). Results indicated that cement injection increased the peak fracture load > 80 and 20% in the simulated fall and one single limb stance configurations, respectively (14). This technique could become a treatment option to solve the problems with osteoporotic hip fractures in patients at risk. Such treatment may hold a significant impact on the economy and human health as hip fractures result in 300,000 hospital admissions annually in the United States with an estimated $9 billion in direct medical costs (14). Therefore, bone cements are significantly valuable for biomedical applications.
In many cases, the bone cemented implants perform satisfactorily for years. However, failure of the implants may be linked to bone cement properties. It is well recognized that there are a number of drawbacks that exist with the usage of bone cement, significant ones including its poor mechanical properties and the potential necrosis of bone tissues (6,15,16). For example, bone cement has been called the ‘‘weak link’’ in the prosthesis system and long-term loosening of the prosthesis has been attributed to its mechanical disintegration (10). It is worth pointing out that aseptic loosening of a cemented arthroplasty is a multifactorial phenomenon involving interfacial failure, bone failure, bone remodeling, and cement failure (6). It is not firmly established whether the drawbacks of bone cements contribute to the initiation or are the consequence of aseptic loosening of the implant (6). In spite of the many drawbacks of bone cement, the survival probabilities of recently cemented joint replacements are still high. Currently, cemented total hip arthroplasty shows survival rates of 90% at 15 years and 80–85% at 20 years (10,15,17–21). Increasing bone cement properties along with improving cementing techniques and implantation methods may further extend cemented implant longevity.
In recognizing the drawbacks of bone cement usage, there have been considerable efforts to secure the implants without using cement. Early noncemented prostheses were the press-fitted or screwed-in types (10). The press-fit
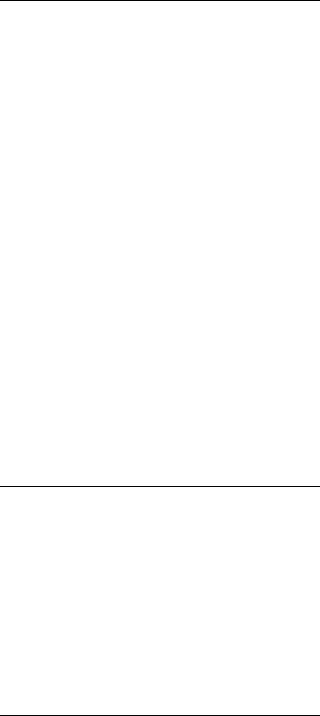
techniques must lead to good initial fixation; otherwise, mobility will occur and this will facilitate wear and/or formation of fibrous tissue. Such techniques have limited successes due to a number of reasons, including bone resorption, geometrical constraints, and increased operational fractures. Noncemented porous coated prostheses were also introduced to provoke bony ingrowth for improved fixation. The noncemented prostheses are used predominantly in younger patients (< 60 years), where it is assumed that these prostheses eventually simplify a revision operation (10). Bone ingrowth strategies are not appropriate for older patients. Research indicates that some of the noncemented devices have failed, but others are doing well in the midto longterm (10). The clinical applications of cementless total hip and knee arthroplasties induce a new set of problems, including perioprosthetic osteolysis, high thigh pain, and failure of the bone–implant interface (6). Thus, there is currently a resurgence of interest in bone cement (6). Definite conclusions about the ultimate clinical performances of cement or cementless fixation devices require longer term studies (10). The debate is still open as to which fixation method is best. The development of cementless modes of fixation is an area of active research and clinical practice; however, acrylic bone cement continues to be the most commonly used nonmetallic implant material by orthopedic surgeons (6,11).
The literature on bone cement is voluminous with respect to its material development, manufacturing, thermal response, chemical and biological effects to bone, and its shortand long-term physical and mechanical properties (static, fatigue, etc.). This article discusses some of these aspects, especially on cement’s material characteristics. For more ample assessment, the readers should refer to other excellent comprehensive reviews [e.g., Krause (3,11), Lewis (6,22), Kuhn (2), Saha (23), Kenny (5), Deb (24), Hasenwinkel (15), Serbetci and Hasirci (16)]. The article is arranged into several sections. It begins with the description of bone cement compositions, followed by a section on cement setting procedure and cementing technique. Then, the thermal and volumetric change effects are discussed. The final section focuses on the physical and mechanical properties of cement. Finally, the article ends with a brief summary.
CEMENT COMPOSITIONS
There are a number of bone cements [> 60 types (2)] available to the orthopedic community. Some popular cements currently available in the United States are given in Table 1 (2,6,11). Most of the present commercial bone cements have similar compositions (Table 2) (2,11,25). The presently used form of bone cement is predominantly the same as the one Sir John Charnley introduced. All acrylic bone cements on the market are chemically based on the identical basic substance: methyl methacrylate (MMA) (2). Pure MMA exhibits a shrinkage of 21% during polymerization (2), and the polymerization temperature can increase to 100–1208C. Such a high shrinkage is intolerable for use in bone cement (2). For this reason, bone cements
|
BONE CEMENT, ACRYLIC |
541 |
Table 1. List of Popular Commercial Bone Cements |
||
Currently Available in the United Statesa |
|
|
Bone Cements |
Manufacturer or Distributor |
|
CMW1 |
Depuy, Warsaw, IN |
|
CMW3 |
Depuy, Warsaw, IN |
|
Palacos R |
Smith and Nephew, Memphis, TN |
|
Simplex P |
Stryker Howmedica Osteonics, |
|
|
Rutherford, NJ |
|
Osteobond |
Zimmer, Warsaw, IN |
|
Zimmer dough-type |
Zimmer, Warsaw, IN |
|
aSee Refs. 2,6, and 11.
are offered as two-component systems in the marketplace: a prepolymerized powder and a liquid mm monomer (2). The MMA in aqueous suspension is prepolymerized in easily cooled reaction boilers. The polymer, obtained in the form of tiny balls (< 150 mm), is easily dissolved in the MMA. By using the prepolymerized polymer powder, both the shrinkage of the sample and the temperature of the reaction can be considerably decreased. In most bone cements on the market, the mixing ratio is two to three parts powder to one part monomer. This reduces the shrinkage and the generation of heat by at least two-thirds, as only the monomer is responsible for these reaction symptoms (2).
Usually, the solid part of bone cement consists of prepolymerized PMMA beads ranging in size from 1 to 150 mm. Other kinds of polymers sometimes are added, including poly(ethyl acrylate), poly(methyl acrylate), poly (styrene), and poly(butyl methacrylate). Free radicals of benzoyl peroxide (BPO) are present within the beads as remnants from the emulsion polymerization process (the process by which most of the beads are manufactured). An additional amount of BPO is mixed with the solid to obtain 1–2.5% by weight benzoyl peroxide. In addition, bone cements generally contain 10–15% by weight barium sulfate, zirconia, or other additive. The presence of barium sulfate or zirconium dioxide in the powder is necessary for
Table 2. Compositions of Commercial Bone Cements Currently Available in the United Statesa,b
Liquid component |
|
Methylmethcrylate (monomer)/ |
98 |
Butylmethacrylate |
|
(binding agent) |
|
Activator/Co-initiator: Dimethyl- |
0.4–2.75 |
paratoluidine |
|
Stabilizer/inhibitor/radical catcher: |
15–75 ppm |
Hydroquinone, Ascorbic acid |
|
Coloring: Chlorophyll |
267 ppm |
Powder |
|
Poly(methyl methacrylate)/copolymer |
90 |
Initiator: Benzoyl peroxide |
0.5–3 |
Opacifier: BaSO4 or ZrO2 |
10–15% |
Coloring: Chlorophyllin |
200 ppm |
Antibiotics: Gentamicin, |
|
erythromycin, and colistin |
|
aCompositions are in percent (w/w) except where stated otherwise. bSee Refs. 2,6,11 and 25.
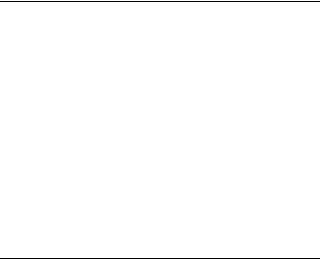
542 BONE CEMENT, ACRYLIC
a clinical reason. The original bone cements, which did not contain radiopacifiers could not be visualized on radiographs. The main components in the liquid phase are MMA, and, in some bone cements, other esters of acrylic acid or methacrylic acid, one or more amines (as activators for the formation of radicals), a stabilizer and, possibly, a colorant (2). The amine in the bone cement, N,N-dimethyl- p-touluidine (DMPT), acts as an accelerator. The liquid component also consists of 50–100 ppm hydroquinone, which inhibits the polymerization reaction within the monomer and allows for storage of the liquid component. Some cement also contains chlorophyll that gives it a green color. This allows better distinction from body tissues during surgery.
Prosthesis-related infection is described as a devastating failure scenario of a cemented orthopedic implant. Infectious complications of a cemented prosthesis lead to a deterioration of function and increase pain. Buchholz and Engelbrecht first reported on the possibilities of mixing antibiotics in bone cement in 1970 (17). They considered gentamicin sulfate to be the antibiotic of choice because of its wide-spectrum antimicrobial activity, its excellent water solubility, its thermal stability and its low allergenicity. Apart from gentamicin, other antibiotics have also been used as an additive to bone cement. The combination of erythromycin and colistin is an example that made it to a commercial product (17). In most cases, the manufacturers make their antibiotic cements by simply mixing antibiotic to a plain cement version they have (2,15,16).
Currently, bone cement fracture is regarded as a major factor in the mechanical failure of implant fixation (26–28). It is directly related to the mechanical properties of the cement, especially the resistance to fracture of the cement in the mantle at the cement–prosthesis interface or the cement–bone interface. Thus, many investigators have attempted to incorporate second phase materials including polyethylene (29), hydroxyapatite (30), PMMA (31), Kevlar (32,33), carbon (34–36), titanium (37), and steel (38–40) to improve the fatigue properties and fracture toughness of the PMMA. Most of the results regarding the properties of these composite materials have been encouraging; however, the biocompatibility issues regarding some of these fibers are as yet unresolved (6). Consequently, none of the commercial bone cements have incorporated these fibers in cements on the market.
CEMENT SETTING AND CEMENTING TECHNIQUE
Most structural materials are fabricated under controlled conditions at a factory, then transported and assembled on site. Bone cement is one of the few structural materials that are created in situ. The surgeon prepares the bone cement directly at the operation table according to the manufacturer’s instructions. All of the cements are supplied in sets of the polymer powder and the monomer liquid components packed in two separate containers within a package. At the time of surgery, the liquid monomer and powder are mixed, the DMPT reacts with the BPO to generate free radicals, which in turn are used in the
Table 3. Four Phases of Bone Cement Polymerization
Processa
|
Time Duration, |
|
Phases |
min |
Characteristics |
|
|
|
I. Mixing |
1–2 |
Wetting |
|
|
Cement relatively liquid |
|
|
(low viscous) |
II. Waiting |
2–3 |
Swelling þ polymerization |
|
|
Increase of viscosity |
|
|
Polymer chains, less |
|
|
movable |
|
|
Sticky dough |
III. Working |
5–8 |
Chain propagation |
|
|
Reduced movability |
|
|
Increase of viscosity |
|
|
Heat generation |
IV. Setting |
2–6 |
Chain growth finished |
|
|
No movability |
|
|
Cement hardened |
|
|
High temperature |
aSee Ref. 2.
additional polymerization of the MMA monomers to form PMMA. Polymer chains from the PMMA become available for free radical polymerization and entanglements of these chains with newly formed chains lead to an intimate connection between the newly formed PMMA with what was already present. The resulting product is a doughy mixture that later polymerizes to a hard and brittle substance.
The curing process of acrylic PMMA bone cement can be divided into four basis steps (2): the mixing, waiting, working, and hardening phase. The characteristics of these phases, well described by Kuhn (2), are shown in Table 3. The time at which the cement does not stick to the surgeon’s glove is referred to as dough time (Fig. 1). The waiting phase ends at this time point. This occurs 2–3 min after the beginning of mixing for most PMMA cements in an ambient temperature of 23 8C (2,11). The working phase is the time during which the surgeon can easily apply cement to the femur. For manual application, the cement must no longer be sticky during this phase, and the viscosity must not to be too high. With the use of mixing systems, the user needs not to wait until the cement is no long sticky. The working time from the end of dough time to the cement is too stiff to manipulate is usually 5–8 min. The cement will fully polymerize to a hardened mass within 8–12 min after initial mixing (2,11).
The quality of the cement dough produced in the operation room will have considerable influence on the clinical long-term result of a cemented prosthesis. Mixing of cements is an important step, as it has a noticeable influence on the mechanical properties of acrylic bone cements (2,6,24). In the 1970s, loosening of the femoral stem was the most common reason for total hip arthroplasy revision, often occurring 5–10 years postoperatively with early component design and cement technique (21). Early methods of cement preparation involved hand mixing in open air of the MMA monomer with the prepolymerized powder mixture. A slurry was formed that could be hand patted or injected into the femoral canal. Many air bubble voids were
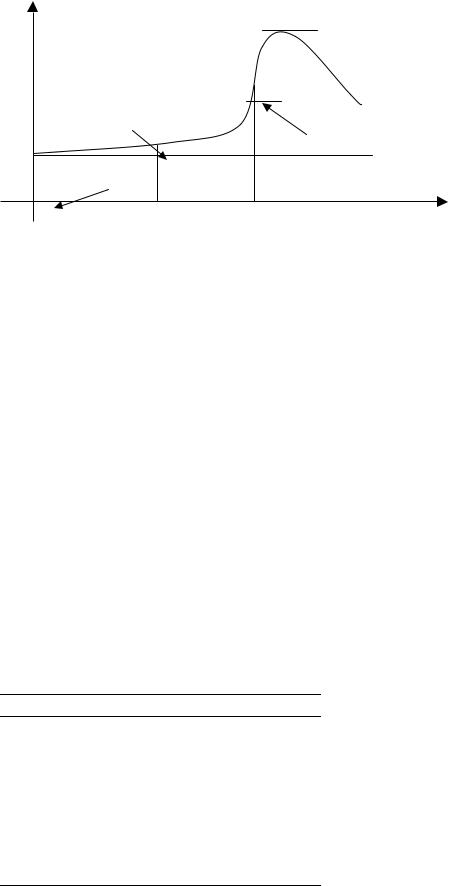
TEMPERATURE |
|
|
|
Tmax |
|
(Tambient + Tmax)/2 |
|
|
Dough time |
|
|
|
Setting time |
Tambient |
|
|
|
Start of mixing |
Working |
|
|
time |
|
0 |
TIME |
|
|
|
BONE CEMENT, ACRYLIC |
543 |
Figure 1. A typical temperature changes with respect to time during cement polymerization. Time zero is designated when the monomer liquid is added to the polymer powder.
created in the mixture during the hand-mixing process. To address this problem in cemented arthroplasty, intensive studies have attempted to improve the technique of cemented stem insertion (21). This results in advancing the cementing techniques from first generation cementing to second and third generation cementing (Table 4). Traditionally, bone cement was mixed using a spatula–bowl arrangement (first generation), which can have the consequence of introducing a high degree of porosity into the cement structure. In addition, the person mixing the cement was exposed to a high level of methyl methacrylate vapors. Second-generation cementing mainly consisted of the use of a canal plug, a cement gun and a high strength cement (21,41,42). Injection guns have been advocated for application of bone cement because long slender injection tips are useful for inserting the cement deep into the cavity, instead of trying to apply it by hand. Gun application also reduces the tendency to form laminations and voids in the cement and also reduces the inclusion of blood into the cement. Substantial improvements in stem survival were reported using such techniques, resulting in stem loosening rates of < 5% at 5–10 years (21,42). The introduction of porosity reducing measures marked the start of third generation cementing techniques. Cracks may initially occur at the voids in bone cements, which act as stress concentration points (24). Vacuum mixing is a typical ways to achieving pore reduction. Other third-generation devel-
Table 4. Developments in Cementing Techniques
Generations |
Techniques |
Time |
1st Generation |
Finger packing |
1960s |
|
No cement restrictor |
|
2nd Generation |
Intramedullary femoral plug |
1970s |
|
Cement gun |
|
|
High-strength cement |
|
3rd Generation |
Pressurization of cement |
mid-1980s– |
|
after insertion |
|
|
Porosity reduction |
|
|
(vacuum mixing) |
|
|
Surface roughening or texturing |
|
|
Precoating |
|
|
Avoiding trochanteric osteotomy |
|
opments in cementing techniques are the use of prosthesis positioning devices that ensure correct placement of the prosthesis, pressurization, and enhanced surface finishes (21,43). Attempts to improve cement-bone interlock using techniques such as endosteal preparation, retrograde cement insertion and cement pressurization improve implant survival. Improving cementing techniques have assisted to dramatically decrease the number of failure in the last three decades. Currently, cemented total hip arthroplasty shows survival rates of 90% at 15 years and 80–85% at 20 years (10,17,18,21).
POLYMERIZATION HEAT
Bone cement generates significant thermal energy during cement setting (an energy of 52 kJ mol 1 of MMA (2)) and this may result in a temperature increase in the cemented system. The exothermic response of the bone cement can be characterized according to American Society for Testing and material (ASTM) standard F451 (44) or international standard ISO 5833. In the ASTM test, a package of cement is mixed at an air conditioned room (23 1 8C, 50 10% relative humidity) as directed by the manufacturer’s instructions. Within 1 min after doughing time, 25 g of the dough is then gently packed into an ASTM specified test mold to achieve a 60 mm diameter with 6 mm thick disk cement mantle (44). The setting curve of temperature change with respect to time (Fig. 1) is recorded with a thermocouple (usually No. 24 gage wire k-type thermocouple) placed at the cement mantle center from the onset of mixing until cooling is observed. The setting time is defined as the time from initial mixing to the time at which the temperature of the polymerising mass has reached half of the maximum temperature (peak temperature) (44). The setting times range from 6 to 14 min and peak temperatures range from 54 to 83 8C for the popular commercial bone cements (2,11). A number of variables will affect the measured setting time and peak temperature, including the powder/liquid ratio of the cement, cement preparing method, cement mantle thickness, the initial temperature of the cement, and the ambient conditions (11). By mixing bone cement with Howmedica Mix-Kit I system and the Zimmer Osteobond vacuum system, Dunne and Orr (45)
544 BONE CEMENT, ACRYLIC
found peak temperatures increased from 36 to 46 8C and 41 to 59 8C for Palacos R and CMW3 bone cement, specifically. Cold cement in a cold room takes longer to set. Precooling cement prior to use extends the cure time and decreases the peak temperature (46). Using a test mold and cementing techniques that simulated a clinical situation, Iesaka et al. (47) showed that the peak temperatures at the bone– cement interface were 53.1, 50.2, and 48.8 8C, while the polymerization time was 4.1, 8.3 and 11.2 min, when the monomer component of bone cement was initially at 37, 23, and 4 8C, respectively. Research also found that bone cement thickness has significant effects on the thermal responses. Meyer et al. (48) found a setting temperature of 60 8C with 3 mm thick specimens of Simplex R, and 107 8C with 10 mm thick specimens. Sih and Connelly (49) showed that the temperatures were 41, 56, and 60 8C for cement thicknesses of 1, 5, and 6-7 mm, respectively. Vallo (50) and Li et al. (51) demonstrated similar results with finite element modeling. Test mold materials also affect the measured setting time and peak temperature (50,51). According to the ASTM methodology, there is no limit to the setting time. However, the maximum allowable temperature for bone cements is 90 8C (44). There are limits stipulated for setting time and doughing time with ISO 5833 Standard.
The importance of temperature rise is that it may result in thermal necrosis of the bone tissue surrounding the implant (2,11,52–56). In vitro studies have shown that maximum temperature of bone cement can be reach higher than 100 8C, varied between 37 and 122 8C in different reports (57). Wang et al. (58) measured four different brands of bone cement (Palaces R, Simplex P, Sulfix, and CMW 1) during polymerization and the peak temperature in the cement was 46–124 8C. Clinical tests show a considerable lower temperature in the body (2,55). The bone-cement interface temperatures have ranged from 35 to 70 8C (2,11,52,3,54,55,57,59). Reasons for this observation are the thin layer of cement ( 3–5 mm) and the blood circulation and heat dissipation in the vital tissue connected with it (2). Moreover, further heat dissipation of the system is attained via the prosthesis (2). Belkoff and Molloy (59) found that peak temperatures at the anterior cortex ranged from 44 to 113 8C, in the center ranged from 49 to 112 8C, and 39 to 57 8C at the spinal canal in the ex vivo vertebroplasaty tests. Toksvig-Larsen (57) performed tests with 31 total hip replacements and showed that the maximum temperature in the acetabulum ranged from 38 to 52 8C and in the femur from 29 to 56 8C. It has been found that not only the temperature, but also the exposure time plays a significant role in direct thermal cell necrosis (52,60–63). The findings of Moritz and Henriques (60) suggest that epithelial cell necrosis occurs 30 s after exposure to a temperature of 55 8C and 5 h after exposure to 45 8C. Lundskog (61) roughly confirmed these trends for bone cells, although he found a slightly lower threshold level. For example, he observed bone cell necrosis after 30 s at 50 8C. He also established that the regenerative capacity of the bone tissue is only damaged after exposure to temperatures of 70 8C and above. Eriksson and Alberksson (64) found the temperature threshold for impaired bone regeneration to be in the range of 44–47 8C for 1 min exposure.
Thermal damage to bone tissue caused by cement polymerization cannot be ruled out and attempts to lower the potential degree of thermal necrosis have been investigated. To reduce the risk of thermal injury it is necessary to avoid exposing bone to temperatures above a certain threshold (62). The thermal responses rely on the quantity of heat produced by the bone cement (cement formulations and cement volume, etc.), rate of heat produced and how the heat conducts (55). The temperature peak can only by influenced to a small extent by adding heat-conducing radiopaque media or by slightly changing the chemical composition of the liquid (2). This, will, however, result in quite different dissolution properties with the polymer, which means it will result in different working properties and, usually, a significant reduction in mechanical stability
(2). The thicker the cement mantle, the greater the volume of material and hence the more heat generated. However, simply reducing the thickness of the mantle is not a favorable option as the mechanics of the joint is affected by this. A slightly reduced level of heat generation has been shown by vacuum mixing the bone cement (45,57). Precooling the cement constituents prior to mixing increases the setting time, but has only minor effects on the maximum temperature of the cement (56). The use of a precooled femoral prosthesis did not affect the peak temperature as well (56,57). To avoid local tissue damage, surgeons may irrigate the implant with ice-cold saline during the polymerization process to decrease both the duration and the level of temperature elevation. Without cooling, the temperature was 49 8C (41–67) at the bone– cement interface, while it decreased to 41 8C (37–47) with water cooling in 19 cases of arthroplasties (65). Investigations on the potential tissue thermal necrosis have obtained varied results: while thermal bone necrosis due to cement curing heat has not been widely reported, some studies showed that thermal necrosis of bone did occur (52,54,59). Nevertheless, few would disagree that a clear understanding of the potential thermal necrosis problem requires further investigations.
VISCOSITY
During the working stage of the cement, its viscosity must be low enough to make it easy to force the cement dough through the delivery system and cause it to flow and penetrate into the interstices of the bone surface in a very short time (6,11,22). In vitro determination of viscosity is usually accomplished with the use of a capillary extrusion or rotational rheometer (6,11,22). The viscosity of the material can be determined by causing the material to flow at a specific shear rate and measuring the shear stress of the fluid against the stationary instrument (11). Viscosity varies across cements. The dynamic viscosity of its dough during the mixing period has been used to categorize bone cement materials into low viscosity brands (e.g., Osteopal), medium-viscosity brands (e.g., Simplex P), and high viscosity brands (e.g., Palacos R) (22). High viscosity bone cements typically have a doughy consistency; low viscosity cements are similar to viscous oil in consistency. Low viscosity cements have a long liquid
phase, or low viscosity wetting phase. The cement remains sticky for quite some time. Viscosity increases rapidly during the working phase, and the doughy cement becomes warm and sets quickly. High and low viscosity bone cements have different handling characteristics and require different cementing techniques. For optimal results, it is necessary to observe the specific mixing instructions for a given bone cement in combination with a given mixing system. Although some researchers suggest that low viscosity brands may have longer fatigue lives compared to high viscosity ones, some report no significant difference (22).
POROSITY, VOLUMETRIC CHANGES, AND RESIDUAL STRESS
Polymerized bone cement is a porous material, containing macropores (pore diameter > 1 mm) and micropores (pore diameter 0.1–1 mm) (6). The degree of porosity varies with cement brands and mixing methods. Jasty et al. (66) found that the porosities were 11.99% (CMW), 9.70% (Palacos R), 9.39% (Simplex P), 12.38% (Zimmer Regular), and 5.00% (Zimmer LVC) using manual mixing, while they were 6.00% (CMW), 4.25% (Simplex P), 6.00% (Zimmer Regular) and 4.15% (Zimmer LVC) with centrifugation. For CMW 1 cement, Muller et al. (67) found that the porosity decreased from 6.67 to 1.28% when using vacuum-mixing instead of hand-mixed methods. The pores generated in polymerized bone cement have been attributed to several sources (6,68–72). It may result from the air that is initially present in the powder interstices; entrapped during blending, mixing, transfer, or delivery; or entrained during insertion of the metal stem. Evaporation of the liquid monomer at the high temperatures of polymerization may contribute. Another aspect of porosity development is polymerization shrinkage.
The presence of pores in the polymerized cement may affect its mechanical properties. Pores may act as stress risers and initiation sites for cracks, rendering the cement susceptible to early fatigue failure (6). However, pores also may play a role in blunting crack propagation, thereby prolonging the life of the implant (73). In vitro experiments, the static and fatigue strength of bone cement both usually decrease with increased porosity (6,22,23,69,70). Reducing the porosity of both bulk cement and its interfaces should be of clinical benefit. Several methods of reducing the porosity of the bone cement have been developed (66). One commonly used method of void reduction is the centrifugation of a chilled, premixed bone cement prior to insertion into the bone. The other one is the mixing of the bone cement in a vacuum environment. Both techniques have been shown to substantially reduce the porosity of bone cement. It has been observed that mixing procedures plays a significant role in determining the quality of bone cement produced (2,74,75). The influence of vacuum mixing on the pores results in a 15–30% improvement of the bending strength of Palacos R while centrifuging Simplex P cement reduced its porosity from 9.4 to 2.9%. Experiments have shown that relative to hand mixing, centrifugation or vacuum mixing leads to a substantial reduction in porosity (2,6). The extent of such a
BONE CEMENT, ACRYLIC |
545 |
reduction depends on many mixing variables, including mixing system, monomer storage temperature, vacuum pressure and centrifugation speed, and durations. In the hand-mixing process, air bubbles are mixed into the dough by thorough mixing. The porosity of the material is high and mechanical stability is endangered. Slower mixing of cement over a shorter time decreases air voids within cement and thus improves the strength characteristics. A high degree of porosity is found to exist in cement that is inadequately mixed (74). Monomer bubbles can easily appear, which may develop during the evaporation of the monomer while evacuating the system or later during polymerization under high pressure by the faulty use of vacuummixing systems.
Cement porosity distributions, especially at the bone– cement interface, may affect the cemented system. There is strong evidence that cracks in the cement are initiated at voids, particularly at the cement–prostheses interface (69). The preferential formation of voids at this site results from shrinkage during bone cement polymerization and the initiation of this process at the warmer bone–cement interface, which causes bone cement to shrink away from the prosthesis (2,68,69). It is expected that a reversal of polymerization direction would shrink the cement onto the prosthesis and reduce or eliminate the formation of voids at this interface (69). One innovative surgical approach to affect this behavior is to preheat the prosthesis prior to implantation. Results indicated that voids near the cement–prosthesis interface decreased significantly (69,76). The porosity at the cement–prosthesis decreased from 16.4 to 0.1% when the prosthesis was preheated to 378C from room temperature, 23 8C. Additionally, the residual stress due to such polymerization curing was shown to decrease significantly at the cement–prosthesis interface (77). Studies also showed that preheating the prosthesis prior to implantation is unlikely to produce significant thermal damage to the bone when compared to implanting a prosthesis initially at room temperature (46,69). However, this procedure may induce more voids at the bone–cement interface, and its effects on the damage at this region should be studied (69,78).
The conversion of the monomer molecules into a polymer network is accompanied with a closer packing of the molecules, which leads to bulk contraction (68,79). A number of devices for determining the volumetric changes have been applied, including using a mercury dilatometer or a water displacement dilatometer (79,80). Theoretical calculations predict that bone cement polymerization will produce a volumetric shrinkage of 8% (81). Muller et al. (67) found volume shrinkages of CMW 1 bone cement were 3.43 and 5.99% with hand and vacuum mixing, respectively. Gilbert et al. (68) found shrinkages of Simplex P cement were 5.09 and 6.67%, respectively. The measured volume shrinkage is less than the theoretical prediction. This can be explained by void growth during polymerization (67).
In a situation where a curing material is bonded on all sides to rigid structures or constrained, bulk contraction cannot occur freely, and shrinkage must be compensated for by some kind of volume generation. This can come from a strain on the material and mainly for dislodgement of the bond, increase in porosity or internal loss of coherence (79).
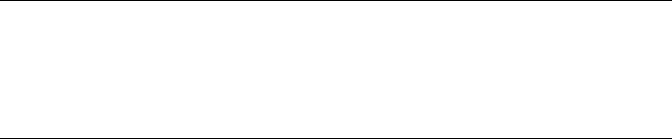
546 BONE CEMENT, ACRYLIC
Table 5. Comparison of the Values of Three Mechanical Properties of Six Different Bone Cements Under Same Test Regimesa
|
|
|
|
DIN53435 |
Impact |
Cement |
ISO 5833 Bending |
Bending |
Compressive |
Bending |
Strength, |
Brands |
Strength, MPa |
Modulus, MPa |
Strength, MPa |
Strength, MPa |
kJ m 2 |
CMW1 |
67.0 |
2634 |
94.4 |
86.2 |
3.7 |
CMW3 |
70.3 |
2764 |
96.3 |
72.4 |
2.9 |
Palacos |
72.2 |
2628 |
79.6 |
87.4 |
7.5 |
Simplex P |
67.1 |
2643 |
80.1 |
70.5 |
3.9 |
Osteobond |
73.7 |
2828 |
104.6 |
80.1 |
3.5 |
Zimmer dough |
62.5 |
2454 |
75.4 |
77.0 |
5.0 |
aSee Ref. 2.
Shrinkage of the polymerizing cement in vivo (i. e., a constrained state) therefore might result in the development of porosity, both at the interfaces and inside the bulk cement. Thus, polymerization shrinkage may be a significant factor in porosity development (68). On the other hand, polymerization may induce high residual stresses. Shrinkage stress occurs when the material contraction is obstructed and the material is rigid enough to resist sufficient plastic flow to compensate for the original volume (79). The process of cement curing is a complex solidification phenomenon where transient stresses are generated and the residual stresses vary with different initial and boundary conditions during curing. A number of approaches have been used to estimate or measure the level of shrinkage stress in bone cement around femoral replacements, including theoretical model, finite element analysis, strain-gage methods and photoelastic methods (52,77,82–88). Currently, the subject of residual stress has often been neglected because it is assumed that residual stress will relax due to the viscoelastic properties of the cement. However, transient and residual stresses are believed to affect cement mechanical responses. Inclusion of the residual stress at the interface resulted in up to a four-fold increase in the von Mises cement stresses compared to the case without residual stresses (83,84). Recently, Orr et al. proposed that residual stresses are sufficient to initiate crack propagation in the cement before any load is applied (85). Lennon and Prendergarst have experimentally observed that residual stresses in the cement may induce cracking even before weight bearing of the cement (89). The initial residual stresses may have immediate effects influencing the possible initiation of cracks and debonding at the cement–prosthesis interface.
MECHANICAL PROPERTIES
Bone cement fills the space between the prosthesis and the bone; this connection is only a mechanical bond (1). Cement mechanical properties are therefore of particular significance for the performance of acrylic bone cement because cement must endure considerable stresses in vivo. There are many physical and mechanical properties of the cement that are considered germane to its clinical performance in the construct, including quasistatic tensile and compressive strength, modulus and ultimate strain, flexural
strength and modulus, shear strength and modulus, fatigue properties (e.g., work of fracture, fracture toughness, fatigue resistance, fatigue crack propagation resistance), and creep (6). The mechanical properties of acrylic bone cement have been widely reported in the literature. Kuhn
(2) investigated the static bending and compressive characteristics of a number of bone cements under the same test regimes (2). Test results of some of the most commonly used bone cement in United States are listed in Table 5. Osteobond cement was shown to have both the highest ISO 5833 bending strength and compressive strength among these six cement brands, while Zimmer dough has both the lowest ISO 5833 bending strength and compressive strength. All the measured bending strengths of the bone cement using the DIN53435 standard were larger than the ones using ISO 5833 standard, with Palacos R bone cement having the highest bending strength in this case. Harper and Bonfield (90) found a wide range of tensile strength (Table 6) and fatigue failure cycles (Table 7) results. They found that the Palacos R and Simplex P cements were significantly higher in tensile strength compared to the other cements tested with exception of CMW 3. There was no statistical difference between the values obtained for CMW 1 and Osteobond. The differences among the fatigue results for the different cements were much larger than those found with the static tensile results. The highest Weibull median fatigue cycles to failure obtained for Simplex P and Palacos R were considerably higher than found for Zimmer dough type. Harper and Bonfield (90) found that there was some correlation between the static and fatigue strengths, but the ranking of static strength does not exactly follow that of fatigue life. The fatigue results were found to correlated well to the clinical data (91): the order of success of implants with the cement brand was the same as that obtained from the fatigue test.
It has long been recognized that PMMA surgical bone cement undergoes viscoelastic (creep) deformation under physiological loads (92,93). Many studies have been performed to assess the viscoelastic properties of bone cement (6,23). Radiological observations of hip stems have shown subsidence of the stem within the cement mantle without visible cement fractures (94). Creep has been implicated in prosthesis subsidence, in particular subsidence of the femoral stem in hip replacements (6,95). Excessive subsidence can lead to prostheses loosening. Lu and McKellop (92) studied the effects of cement creep on the subsidence of
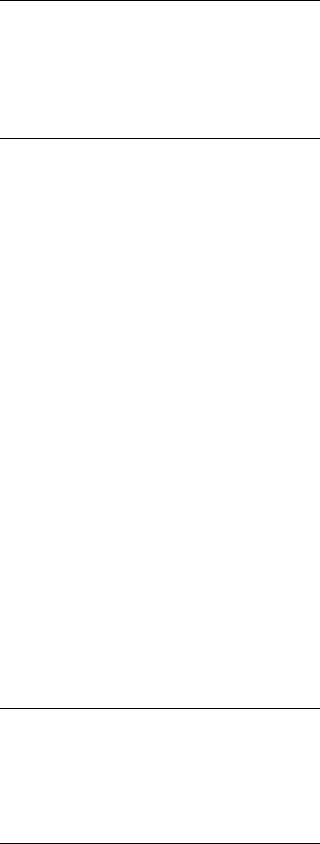
Table 6. Comparison of Tensile of Six Different Bone
Cementsa
|
|
Modulus of |
Strain at |
Cement |
Ultimate |
Elasticity, |
Fracture, |
Brands |
Strength, MPa |
GPa |
% |
|
|
|
|
CMW1 |
39.1 |
2.96 |
1.60 |
CMW3 |
44.7 |
3.53 |
1.36 |
Palacos |
51.4 |
3.21 |
2.25 |
Simplex P |
50.1 |
3.43 |
1.87 |
Osteobond |
38.2 |
3.38 |
1.41 |
Zimmer dough |
31.7 |
2.79 |
1.43 |
aSee Ref. 90.
the stem and on the stress within the cement using a cyclic load and three interface bonding conditions (bonded, frictional, and debonded). Results showed that the creep deformation of the cement was accompanied by additional subsidence of the stem and a decrease in the stress components within the cement. The results agreed with an experimental study using stems cemented into cadaver femora (96). Cement creep would accumulate for the frictional stem–cement interface, resulting in 0.46 mm total stem subsidence and a 13% decrease in the stress within the cement (92). Ling et al. (97) concluded that, at least for smooth tapered stems, that substantial hoop and radial creep of the cement not only occurs, but also is essential for the optimum clinical performance of the prosthesis. On the other hand, a limited degree of creep may help in maintaining the cement–implant interface. Also, Harris (98) stated that creep of the cement mantle surrounding a hip prosthesis may be negligible under cyclic physiological loading. The role of creep of the bone cement on the chance of failure of the cement mantle is still a subject of controversy. Due to its viscoelastic nature, cement tested at different strain rates may have changing characteristics (11). There is still lack of tests of bone cement in ways that represent real-life loading patterns that mimic those experienced by the cement in vivo (99). The true understanding of the mechanical behavior of bone cement can only be attained if the testing procedure is truly representative (99).
There are considerable differences between the values of physical and mechanical properties of bone cement reported in the literature. This disagreement may be the result of cement type, cement preparation technique, specimen geometry, measurement technique, test parameters, and
Table 7. Comparison of Fatigue Test Results of Six Different Bone Cementsa
|
Cycles to Failure |
|
|
|
|
Cement Brands |
Range |
Weibull Median |
|
|
|
CMW1 |
3042–8835 |
4407 |
CMW3 |
5996–38262 |
16441 |
Palacos |
18362–49285 |
27892 |
Simplex P |
8933–93345 |
36677 |
Osteobond |
5527–25825 |
16162 |
Zimmer dough |
153–3978 |
781 |
aSee Ref. 90.
BONE CEMENT, ACRYLIC |
547 |
testing environment (11). Therefore it may be impossible to compare results from different investigations (90). However, results with specific conditions may be found in a number of the excellent comprehensively reviews (1– 3,6,11,22,23). It has been shown that cement formulations play a significant role on the cement mechanical properties (Table 5). Also, the test methods will affect the values obtained, which can be easily seen by comparing the bending strength results using ISO 5833 standard to that using DIN 53435 standard (Table 5). Cement mixing methods (hand mixing, vacuum mixing, or centrifugation) have been shown to affect the physical properties of bone cement (74,100,101). Even with vacuum mixing, using different vacuum mixing systems have resulted in different bone cement porosity, static strength, and fatigue strength (75,102). The storage temperature of cement constitutive was shown to have minor effects on porosity and fatigue performance (100). Ishihara et al. (103) showed that the fatigue of bone cements at 1 Hz are shorter by one to two order of magnitude as compared with fatigue lives at 20 Hz. On the other hand, Lewis et al. (104) found that frequency (over the range used) did not exert a statistically significant effect on the fatigue life of cement tested in their investigations.
SUMMARY
Acrylic bone cement has been widely used in orthopedic surgery. Currently, the cement is not without its drawbacks as discussed previously. One major research area focusing on modifications of cement formulations may lead to obtain more favorable cement mechanical properties and biological compatibilities. One example of these developments is to incorporate second phase materials (bioactive agents, reinforcement fibers, etc.) into the existing bone cement. Another approach to overcome cement weakness is to manipulate cement processing procedure and the surgical techniques. A good example is to reduce cement porosity by vacuum mixing techniques. It is worth pointing out that considerable research is needed to develop techniques for accurate characterizations of cement properties, monitoring cement curing process and evaluations of potential bone cement failure.
BIBLIOGRAPHY
Cited References
1.Walenkamp G, Murray DW. Bone cements and cementing technique. Berlin Heidelberg New York: Springer-Verlag; 2001.
2.Kuhn KD. Bone cement: up-to-date comparison of physical and chemical properties of commercial materials. Berlin: Springer-Verlag; 2000.
3.Krause W, Mathis RS. Fatigue properties of acrylic bone cements - review of the literature. J Biomed Mater Res-Appl Biomate 1988;22(A1):37–53.
4.Charnley J. Anchorage of the femoral head prosthesis to the shaft of the femur. J Bone Joint Surg 1960;43B:28– 30.
548BONE CEMENT, ACRYLIC
5.Kenny SM, Buggy M. Bone cements and fillers: A review. J Mater Sci-Mater Med 2003;14(11): p 923–938.
6.Lewis G. Properties of acrylic bone cement: State of the art review. J Biomed Mater Res 1997;38(2):155–182.
7.NIH. Total hip replacement. NIH Consensus Statement 1994;12(5):1–31.
8.Cristofolini L. A critical analysis of stress shielding evaluation of hip prostheses. Crit Rev Biomed Eng 1997;25(4– 5):409–483.
9.Chao E. Orthopaedic Biomechanics. Int Orthop (SICOT) 1996;20:239–243.
10.Huiskes R, Verdonschot N. Biomech Art Joints: the Hip, Basic Orthopaedic Biomechanics. In: Mow VC, Hayes WC, editors. Philadelphia: Lippincott-Raven Publishers; 1997. 395–460.
11.Krause WR. Bone Cement, Acrylic. In: Webster JG, editor. Encyclopedia of Medical Devices and Instrumentation. New York: John Wiley & sons Inc.; 1988. p 491–500.
12.Wilcox RK. The biomechanics of vertebroplasty: a review. Proceedings of the Institution of Mechanical Engineers Part H. J Eng Med 2004;218(H1):1–10.
13.Phillips FM. Minimally invasive treatments of osteoporotic vertebral compression fractures. Spine 2003;28(15):S45–S53.
14.Heini PF, et al. Femoroplasty-augmentation of mechanical properties in the osteoporotic proximal femur: a biomechanical investigation of PMMA reinforcement in cadaver bones. Clin Biomech 2004;19(5):506–512.
15.Hasenwinkel J. Bone Cement. In: Wnek GE, Bowin GL, editors. Encyclopedia of Biomaterials and Biomedical Engineering. New York: Marcel Dekker; 2004. p 170–179.
16.Serbetci K, Hasirci N. Recent developments in bone cements. In: Yaszemski MJ et al. editors. Biomaterials in Orthopedics. New York: Marcel-Dekker; 2004. p 241–286.
17.Hendriks JGE, et al. Backgrounds of antibiotic-loaded bone cement and prosthesis-related infection. Biomaterials 2004;25(3):545–556.
18.Murray DW, Carr AJ, Bulstrode CJ. Which Primary Total Hip-Replacement. J Bone Joint Surg-Br Vol 1995;77B(4): 520–527.
19.Nafei A, et al. Survivorship analysis of cemented total condylar knee arthroplasty: a long-term follow-up report on 348 cases. J Arthroplasty 1996;11:7–10.
20.El-Warrak AO, et al. A review of aseptic loosening in total hip arthroplasty. Veterin Compar Orthopae Traumatol 2001;14(3):115–124.
21.Barrack RL. Early failure of modern cemented stems. J Arthroplasty 2000;15(8):1036–1050.
22.Lewis G. Fatigue testing and performance of acrylic bonecement materials: State-of-the-art review. J Biomed Mater Res Part B-Appl Biomater 2003;66B(1):457–486.
23.Saha S, Pal S. Mechanical-Properties of Bone-Cement-a Review. J Biomed Mater Res 1984;18(4):435–462.
24.Deb S. A review of improvements in acrylic bone cements. J Biomater Appl 1999;14(1):16–47.
25.Passuti N, Gouin F. Antibiotic-loaded bone cement in orthopedic surgery. Joint Bone Spine 2003;70(3):169–174.
26.Jasty M, et al. The Initiation of Failure in Cemented Femoral Components of Hip Arthroplasties. J Bone Joint Surg Br Vol 1991;73(4):551–558.
27.Hertzberg RW, Manson JA. Fatigue of engineering plastics. London: Academic Press; 1980.
28.Spector M. Biomaterial failure. Orthoped Clin N Am 1992;23(2):211–217.
29.Pourdeyhimi B, Wagner HD. Elastic and Ultimate Properties of Acrylic Bone-Cement Reinforced with Ultra-High-Molecu- lar-Weight Polyethylene Fibers. J Biomed Mater Res 1989;23(1):63–80.
30.Harper EJ, Behiri JC, Bonfield W. Flexural and fatigue properties of a bone cement based upon polyethylmethacrylate and hydroxyapatite. J Mat Sci Mat Med 1995;6:799–803.
31.Gilbert JL, Net SS, Lauthenschlager EP. Self-reinforced composite poly(methylmethacryate): static and fatigue properties. Biomaterials 1995;16:1043–1055.
32.Pourdeyhimi B, Wagner HD, Schwartz P. A Comparison of Mechanical-Properties of Discontinuous Kevlar-29 Fiber Reinforced Bone and Dental Cements. J Mater Sci 1986;21(12):4468–4474.
33.Wright TM, Trent PS. Mechanical-Properties of Aramid Fiber-Reinforced Acrylic Bone Cement. J Mater Sci 1979;14(2):503–505.
34.Saha S. Strain-rate dependence of the compressive properties of normal and carbon-fiber-reinforced bone-cement. J Biomed Mater Res 1983;17(6):1041–1047.
35.Saha S, et al. Biomechanical Evaluation of Bony Defects Repaired with Normal, Carbon-Fiber, and Wire Reinforced Bone-Cement. Biomater Med Devices Artif Organs 1981;9(4):291–291.
36.Pilliar RM, et al. Carbon Fiber-Reinforced Bone Cement in Orthopedic Surgery. J Biomed Mater Res 1976;10(6):893– 906.
37.Topoleski LDT, Ducheyne P, Cuckler JM. The FractureToughness of Titanium-Fiber-Reinforced Bone-Cement. J Biomed Mater Res 1992;26(12):1599–1617.
38.Saha S, Kraay MJ. Bending Properties of Wire-Reinforced Bone-Cement for Applications in Spinal Fixation. J Biomed Mater Res 1979;13(3):443–457.
39.Kotha SP, et al. Fracture toughness of steel-fiber-reinforced bone cement. J Biomed Mater Res Part A 2004;70A(3):514– 521.
40.Fishbane BM, Pond RB. Stainless steel fiber reinforcement of polymethylmethacrylate. Clin Orthop Rel Res 1977;128:194– 199.
41.Mulroy WF, Harris WH. Revision total hip arthroplasty with use of so-called second-generation cementing techniques for aseptic loosening of the femoral component—A fifteen-year- average follow-up study. J Bone Joint Surg—Am Vol 1996;78A(3):325–330.
42.Mulroy WF, Estok DM, Harris WH. Total hip arthroplasty with use of so-called second-generation cementing techni- ques—A fifteen-year-average follow-up study. J Bone Joint—Am Vol 1995;77A(12):1845–1852.
43.Faulkner A, et al. Effectiveness of hip prostheses in primary total hip replacement: a critical review of evidence and an economic model. Health Technol Asess 1998;2(6):1–146.
44.ASTM, ASTM Standard Specification for Acrylic Bone Cement F451-99a; 1999.
45.Dunne NJ, Orr JF. Curing characteristics of acrylic bone cement. J Mater Sci Mater Med 2002;13(1):17–22.
46.Li CD, Schmid S, Mason J. Effects of pre-cooling and preheating procedures on cement polymerization and thermal osteonecrosis in cemented hip replacements. Med Eng Phys 2003;25(7):559–564.
47.Iessaka K, Jaffe WL, Kummer FL. Effects of the initial temperature of acrylic bone cement liquid monomer on the properties of the stem-cement interface and cement polymerization. J Biomed Mater Res: Appl Biomater 2003;68B:186– 190.
48.Meyer PR, Lautenschlager EP, Moore BK. On the setting properties of acrylic bone cement. J Bone Joint Surg 1973;55A:139–156.
49.Sih GC, Connelly GM, Berman AT. The Effect of Thickness and Pressure on the Curing of Pmma Bone-Cement for the Total Hip-Joint Replacement. J Biomech 1980;13(4): 347–352.
50.Vallo CL. Theoretical prediction and experimental determination of the effect of mold characteristics on temperature and monomer conversion fraction profiles during polymerization of a PMMA-based bone cement. J Biomed Mater Res (Appl Biomater) 2002;63:627–642.
51.Li CD, Mason J, Yakimicki D. Thermal characterization of PMMA-based bone cement curing. J Mater Sci—Mater Med 2004;15(1):85–89.
52.Huiskes R. Some fundamental aspects of human joint replacement. Acta Orthopaed Scand 1980; (Suppl.) 185.
53.Mjoberg B. Fixation and Loosening of Hip Prostheses—a Review. Acta Orthopaed Scand 1991;62(5):500–508.
54.Mjoberg B, et al. Bone-Cement, Thermal-Injury and the Radiolucent Zone. Acta Orthopaed Scand 1984;55(6):597– 600.
55.Dipisa JA, Sih GS, Berman AT. Temperature Problem at Bone-Acrylic Cement Interface of Total Hip-Replacement. Clin Orthopae Relat Res 1976;121:95–98.
56.Swenson LW, Schurman DJ, Piziali RL. Finite element temperature analysis of a total hip replacement and measurement of PMMA curing temperatures. J Biomed Mater Res 1981;15:83–96.
57.Toksviglarsen S, Franzen H, Ryd L. Cement Interface Temperature in Hip-Arthroplasty. Acta Orthopaed Scand 1991;62(2):102–105.
58.Wang JS, Franzen H, Toksviglarsen S. Does vacuum mixing of bone-cement affect heat-generation - analysis of 4 cement brands. J Appl Biomater 1995;6(2):105–108.
59.Belkoff SM, Molloy S. Temperature measurement during polymerization of polymethylmethacrylate cement used for vertebroplasty. Spine 2003;28(14):1555–1559.
60.Moritz AR, Henriques FC. The relative importance of time and surface temperature in the causation of cutaneous burns. Am J Pathol 1947;23:695–720.
61.Lundskog J. Heat and bone tissue: an experimental investigation of the thermal properties of bone and threshold levels for thermal injury. Scand J Plastic Reconstr Surg 1972;9:1– 80.
62.Revie I, Wallace M, Orr J. The effect of PMMA thickness on thermal bone necrosis around acetabular sockets. Proc Instn Mech Eng 1994;208:45–51.
63.Nelson C, Krishnan E, Neff J. Consideration of physical parameters to predict thermal necrosis in acrylic cement implants at the site of giant cell tumors of bone. Med Phys 1986;13(4):462–488.
64.Eriksson AR, Albreksson T. Temperature threshold levels for heat induced bone tissue injury: a vital microscopic study in the rabbit. J Prosthetic Den 1983;50(1):101–107.
65.Wykman AGM. Acetabular Cement Temperature in Arthroplasty - Effect of Water Cooling in 19 Cases. Acta Orthopaed Scand 1992;63(5):543–544.
66.Jasty M, et al. Porosity of various preparations of acrylic bone cements. Clin Orthop Rel Res 1990;259:122–129.
67.Muller SD, Green SM, McCaskie AW. The dynamic volume changes of polymerising polymethy methacrylate bone cement. Acta Orthopaed Scand 2002;73(6):684–687.
68.Gilbert JL, et al. A theoretical and experimental analysis of polymerization shrinkage of bone cement: A potential major source of porosity. J Biomed Mater Res 2000;52(1): 210–218.
69.Bishop NE, Ferguson S, Tepic S. Porosity reduction in bone cement at the cement-stem interface. J Bone Joint Surg-Br Vol 1996;78B:349–356.
70.James SP, et al. A fractographic investigation of PMMA bone cement focusing on the relationship between porosity reduction and increased fatigue life. J Biomed Mater Res 1992;26(5):651–662.
BONE CEMENT, ACRYLIC |
549 |
71.James SP, et al. Extensive porosity at the cement-femoral prosthesis interface: A preliminary study. J Biomed Mater Res 1993;27:71–78.
72.Wixson RL, Lautenschlager EP, Novak MA. Vacuum mixing of acrylic bone cement. J Arthroplasty 1987;2:141–149.
73.Topoleski LDT, Ducheyne PI, Cuckler JM. Microstructural pathway of fracture in poly(methyl methacrylate) bone cement. Biomaterials 1993;14(15):1165–1172.
74.Dunne NJ, Orr JF. Influence of mixing techniques on the physical properties of acrylic bone cement. Biomaterials 2001;22(13):1819–1826.
75.Mau H, et al. Comparison of various vaccum mixing system and bone cements as regards reliability, porosity and bending stength. Acta Orthopaed Scand 2004;75(2):160–172.
76.Iessaka K, Jaffe WL, Kummer FJ. Effects of preheating of hip prosthesis on the stem-cement interface. J Bone Joint Surg— Am Vol 2003;85:421–427.
77.Li CD, Wang Y, Mason J. The effects of curing history on residual stresses in bone cement during hip arthroplasty. J Biomed Mater Res Part B-App Biomater 2004;70B(1):30–36.
78.Race A, et al. Early cement damage around a femoral stem is concentrated at the cement/bone interface. J Biomech 2003;36:489–496.
79.Davidson CL, Feilzer AJ. Polymerization shrinkage and polymerization shrinkage stress in polymer-based restoratives. J Den 1997;25(6):435–440.
80.Davies JP, Harris WH. Comparison of diametral shrinkage of centrifuged and uncentrifuged Simplex P bone cement. J Appl Biomater 1995;6:209–211.
81.Hass S, Brauer G, Dickson G. A characterization of polymethyl methacrylate bone cement. J Bone Joint Surg—Am Vol 1975;57:380–391.
82.Ahmed AM, et al. Transient and residual stresses and displacements in self-curing bone cement - part I: characterization of relevant volumetric behavior of bone cement. J Biomech Eng—Trans ASME 1982;104:21–27.
83.Nuno N, Amabili M. Modeling debonded stem-cement interface for hip implants: effect of residual stresses. Clin Biomech 2002;17:41–48.
84.Nuno N, Avanzolini G. Residual stresses at the stem-cement interface of an idealized cemented hip stem. J Biomech 2002;35:849–852.
85.Orr JF, Dunne NJ, Quinn JC. Shrinkage stresses in bone cement. Biomaterials 2003;24(17):2933–2940.
86.Ahmed AM, et al. Transient and residual stresses and displacements in self-curing bone cement-Part II: thermoelastic analysis of the stem fixation system. J Biomech Eng—Trans Asme 1982;104:28–37.
87.Roques A, et al. Quantitative measurement of the stresses induced during polymerization of bone cement. Biomaterials 2004;25:4415–4424.
88.Zor M, Kucuk M, Aksoy S. Residual stress effects on fracture energies of cement-bone and cement-implant interfaces. Biomaterials 2002;23:1595–1601.
89.Lennon AB, Prendergast PJ. Residual stress due to curing can initiate damage in porous bone cement: experimental and theoretical evidence. J Biomech 2002;35:311–321.
90.Harper EJ, Bonfield W. Tensile characteristics of ten commercial acrylic bone cements. J Biomed Mater Res 2000;53(5):605–616.
91.Malchau H, Herberts P. Prognosis of total hip replacement and revision rate in THR: A revision risk study of 148,359 primary operations. 65th Ann Meet Am Acad Ortho Surg 1998. New Orleans.
92.Lu Z, Mckellop H. Effects of cement creep on stem subsidence and stress in the cement mantle of a total hip replacement. J Biomed Mater Res 1997;34:221–226.