
- •VOLUME 1
- •CONTRIBUTOR LIST
- •PREFACE
- •LIST OF ARTICLES
- •ABBREVIATIONS AND ACRONYMS
- •CONVERSION FACTORS AND UNIT SYMBOLS
- •ABLATION.
- •ABSORBABLE BIOMATERIALS.
- •ACRYLIC BONE CEMENT.
- •ACTINOTHERAPY.
- •ADOPTIVE IMMUNOTHERAPY.
- •AFFINITY CHROMATOGRAPHY.
- •ALLOYS, SHAPE MEMORY
- •AMBULATORY MONITORING
- •ANALYTICAL METHODS, AUTOMATED
- •ANALYZER, OXYGEN.
- •ANESTHESIA MACHINES
- •ANESTHESIA MONITORING.
- •ANESTHESIA, COMPUTERS IN
- •ANGER CAMERA
- •ANGIOPLASTY.
- •ANORECTAL MANOMETRY
- •ANTIBODIES, MONOCLONAL.
- •APNEA DETECTION.
- •ARRHYTHMIA, TREATMENT.
- •ARRHYTHMIA ANALYSIS, AUTOMATED
- •ARTERIAL TONOMETRY.
- •ARTIFICIAL BLOOD.
- •ARTIFICIAL HEART.
- •ARTIFICIAL HEART VALVE.
- •ARTIFICIAL HIP JOINTS.
- •ARTIFICIAL LARYNX.
- •ARTIFICIAL PANCREAS.
- •ARTERIES, ELASTIC PROPERTIES OF
- •ASSISTIVE DEVICES FOR THE DISABLED.
- •ATOMIC ABSORPTION SPECTROMETRY.
- •AUDIOMETRY
- •BACTERIAL DETECTION SYSTEMS.
- •BALLOON PUMP.
- •BANKED BLOOD.
- •BAROTRAUMA.
- •BARRIER CONTRACEPTIVE DEVICES.
- •BIOCERAMICS.
- •BIOCOMPATIBILITY OF MATERIALS
- •BIOELECTRODES
- •BIOFEEDBACK
- •BIOHEAT TRANSFER
- •BIOIMPEDANCE IN CARDIOVASCULAR MEDICINE
- •BIOINFORMATICS
- •BIOLOGIC THERAPY.
- •BIOMAGNETISM
- •BIOMATERIALS, ABSORBABLE
- •BIOMATERIALS: AN OVERVIEW
- •BIOMATERIALS: BIOCERAMICS
- •BIOMATERIALS: CARBON
- •BIOMATERIALS CORROSION AND WEAR OF
- •BIOMATERIALS FOR DENTISTRY
- •BIOMATERIALS, POLYMERS
- •BIOMATERIALS, SURFACE PROPERTIES OF
- •BIOMATERIALS, TESTING AND STRUCTURAL PROPERTIES OF
- •BIOMATERIALS: TISSUE-ENGINEERING AND SCAFFOLDS
- •BIOMECHANICS OF EXERCISE FITNESS
- •BIOMECHANICS OF JOINTS.
- •BIOMECHANICS OF SCOLIOSIS.
- •BIOMECHANICS OF SKIN.
- •BIOMECHANICS OF THE HUMAN SPINE.
- •BIOMECHANICS OF TOOTH AND JAW.
- •BIOMEDICAL ENGINEERING EDUCATION
- •BIOSURFACE ENGINEERING
- •BIOSENSORS.
- •BIOTELEMETRY
- •BIRTH CONTROL.
- •BLEEDING, GASTROINTESTINAL.
- •BLADDER DYSFUNCTION, NEUROSTIMULATION OF
- •BLIND AND VISUALLY IMPAIRED, ASSISTIVE TECHNOLOGY FOR
- •BLOOD BANKING.
- •BLOOD CELL COUNTERS.
- •BLOOD COLLECTION AND PROCESSING
- •BLOOD FLOW.
- •BLOOD GAS MEASUREMENTS
- •BLOOD PRESSURE MEASUREMENT
- •BLOOD PRESSURE, AUTOMATIC CONTROL OF
- •BLOOD RHEOLOGY
- •BLOOD, ARTIFICIAL
- •BONDING, ENAMEL.
- •BONE AND TEETH, PROPERTIES OF
- •BONE CEMENT, ACRYLIC
- •BONE DENSITY MEASUREMENT
- •BORON NEUTRON CAPTURE THERAPY
- •BRACHYTHERAPY, HIGH DOSAGE RATE
- •BRACHYTHERAPY, INTRAVASCULAR
- •BRAIN ELECTRICAL ACTIVITY.
- •BURN WOUND COVERINGS.
- •BYPASS, CORONARY.
- •BYPASS, CARDIOPULMONARY.
218.Lowery C, Robinson S, Eswaran H, V. J, H. G, Cheung T. Detection of the transient and steady-state auditory evoked responses in the human fetus. In: Yoshimoto T, Kotani M, Kuriki S, Karibe H, Nakasato N, editors. Recent Advances in Biomagnetism. Sendai: Tohoku University Press; 1999. p 963–966.
219.Rose D, Eswaran H. Spontaneous neuronal activity in fetuses and newborns. Exp Neurol, in press.
220.Eswaran H, Wilson J, Preissl H, Robinson S, Vrba J, Murphy P, Rose D, Lowery C. Magnetoencephalographic recordings of visual evoked brain activity in the human fetus. Lancet 2002;360:779–780.
221.Vrba J, Robinson SE, McCubbin J, Murphy P, Eswaran H, Wilson JD, Preissl H, Lowery CL. Human fetal brain imaging by magnetoencephalography: verification of fetal brain signals by comparison with fetal brain models. Neuroimage 2004;21:1009–1020.
222.Paulson DN, Fagaly RL, Toussaint RM, Fischer F. Biomagnetic susceptometry with SQUID instrumentation. IEEE Trans Mag 1991;27:3249–3252.
223.Brittenham GM, Sheth S, Allen CJ, Farrell DE. Noninvasive
methods for quantitative assessment of transfusional iron overload in sickle cell disease. Semin Hematol 2001; 38: 37–56.
224.Hoshiyama M, Kakigi R, Nagata O. Peripheral nerve conduction recorded by a micro gradiometer system (microSQUID) in humans. Neurosci Lett 1999;272:199–202.
225.Mackert BM, Curio G, Burghoff M, Trahms L, Marx P. Magnetoneurographic 3D localization of conduction blocks in patients with unilateral S1 root compression. Electroencephalogr Clin Neurophysiol 1998;109:315–320.
226.Le Gros V, Lemaigre D, Suon C, Pozzi JP, Liot F. Magnetopneumography: a general review. Eur Respir J 1989;2: 149–159.
227.Huvinen M, Oksanen L, Kalliomaki K, Kalliomaki PL, Moilanen M. Estimation of individual dust exposure by magnetopneumography in stainless steel production. Sci Total Environ 1997;199:133–139.
228.Moller W, Barth W, Kohlhaufl M, Haussinger K, Stahlhofen W, Heyder J. Human alveolar long-term clearance of ferromagnetic iron oxide microparticles in healthy and diseased subjects. Exp Lung Res 2001;27:547–568.
229.Moraes R, Toncon LE, Baffa O, Oba-Kunyioshi AS, Wakai RT, Leuthold AC. Adaptive, autoregressive spectral estimation for analysis of electrical signals of gastric origin. Physiol Meas 2003;24:91–106.
230.Kosch O, Osmanoglou E, Hartman V, Strenzke A, Weitschies W, Wiedenmann B, Monnikes H, Trahms L. Investigation of gastrointestinal transport by magnetic marker localization. Biomed Tech (Berl) 2002;47(Suppl 1, Pt 2): 506–509.
See also ELECTROCARDIOGRAPHY, COMPUTERS IN; ELECTROENCEPHALOGRAPHY; EVOKED POTENTIALS; PULMONARY PHYSIOLOGY.
BIOMATERIALS, ABSORBABLE
MARK BORDEN
Director of Biomaterials
Research
Irvine, California
INTRODUCTION
Historically, the use of implants in orthopedic surgery has originated from fracture repair and joint replacement
BIOMATERIALS, ABSORBABLE |
255 |
applications. During the late 1920s, stainless-steel bone implants such as Kirshner nails and Steinman pins were popularized for the surgical treatment of fractures (1). With the introduction of new surgical materials such as cobalt alloys, polyethylene and poly(tetrafluoroethylene) [Teflon], surgeons and engineers began working toward the design and fabrication of artificial joints. The advent of new high strength implant materials allowed researchers such Dr. John Charnley to begin pioneering work in total hip replacement surgery in the late 1930s (1,2). As advances in chemistry, metallurgy, and ceramics progressed throughout the years, a large variety of implants have entered the orthopedic market. Today, orthopedic implants are composed of specialized metals, ceramics, polymers, and composites that possess a large range in properties. Although these materials have been successfully fabricated into a variety of implants, one common issue has remained. Once the device has performed its required function and is no longer needed, it remains as a bystander in the now healthy tissue. The issue is that the long-term presence of an implant in the body can result in implant-related complications such as loosening, migration, mechanical breakdown and fatigue, generation of wear particles, and other negative effects (3–6). With prolonged patient life spans and higher activity levels, more and more people are now outliving the lifetime of their implants.
The potential for long-term implant problems has driven researchers to look to a unique category of materials that are capable of being completely resorbed by the body. These bioresorbable or biodegradable materials are characterized by the ability to be chemically broken down into harmless byproducts that are metabolized or excreted by the body. Materials of this type offer a great advantage over conventional nonresorbable implant materials. Bioresorbable implants provide their required function until the tissue is healed, and once their role is complete, the implant is completely resorbed by the body. The end result is healthy tissue with no signs that an implant was ever present. As the implant is completely gone from the site, long-term complications associated with nonresorbable devices do not exist.
ORTHOPEDIC APPLICATIONS OF RESORBABLE IMPLANTS
The ability of a resorbable implant to provide temporary fixation followed by complete resorption is a desirable property for a large variety of surgical applications. In relation to orthopedic surgery, this behavior is particularly useful based on the goal of restoring physiological function to the tissues and joints of the skeleton. In general, orthopedic surgery is often compared with carpentry in that the surgeon’s instruments often consist of hammers, drills, and saws. Similar to carpentry, specialized screws, plates, pins, and nails are used to fix one material to another. In orthopedics, this fixation can be categorized into two main areas: bone-to-bone fixation and soft tissue-to-bone fixation. Bone fixation is used in the treatment of complex fractures and in reconstructive procedures of the skeleton. The implants used in these surgeries are designed to

256 BIOMATERIALS, ABSORBABLE
maintain the position of the bone fragments, to stabilize the site, and to allow for eventual fusion of the fracture. As a result of the fracture healing process, the bone is remodeled so effectively that it is often difficult to locate the initial injury. With nonresorbable implants, the long-term presence of the device only serves as a source for potential complications. Resorbable implants, on the other hand, alleviate this concern by fully resorbing and allowing the bone to completely remodel into its normal physiological state.
In addition to bone fixation, soft tissue fixation is also an excellent application of resorbable implants. This type of reconstruction is often the result of trauma to joints such as the knee and shoulder. Typically developing from sports injuries or accidents, the goal is to restore stability to the joint by replacing or reconstructing the ligament or tendon interface to bone. In the knee, for example, the reconstruction of a torn anterior cruciate ligament (ACL) is a common sports medicine procedure. This type of surgical reconstruction consists of replacing the torn ACL with a bone- tendon-bone graft taken from the patient’s patella and fixing the graft across the joint. During the procedure, the bony portion of the ACL graft is fixed in bone tunnels drilled into the tibia and femur. In order to stabilize the graft and aid in the formation of a stable bone-to-ligament interface, interference screws are used to fix the graft to the site. Once bone has been incorporated into the graft, the device is no longer needed.
Another example of soft tissue reconstruction is the repair of a tear in the rotator cuff tendon of the shoulder. This type of injury requires reestablishing the tendon- to-bone interface. To facilitate this process and restore stability to the shoulder, implants called suture anchors are used to provide a means to affix the torn tendon to the bone of the humerous. Just as the name describes, these implants function by providing an anchor in bone that allows the attached suture to tighten down on the tendon and pull it in contact with bone. As healing progresses, a stable interface develops and joint function is restored. Similar to other fixation applications, once the interface has fully healed, the implant is no longer needed.
FUNCTION OF A RESORBABLE IMPLANT
As seen from the various types of tissue fixation procedures within orthopedic surgery, resorbable implants are exposed to a variety of healing environments. Out of the currently used materials in orthopedic surgery, only the polymer and ceramic groups contain resorbable biomaterials. It is the specific properties of these materials that allow them to be used as resorbable devices. In evaluating a material for potential use as an implant, the key properties include implant biocompatibility, resorbability, and mechanical properties. The first criteria, biocompatibility, refers to the ability of the material to be implanted into the body without negatively affecting the surrounding tissue, which includes the absence of inflammation, toxicity (materials that kill surrounding cells), carcinogenicity (materials that can cause cancer), genotoxicicty (materials that damage the DNA of sur-
Implant |
Tissue |
Mechanical properties
Time
Figure 1. Optimal stress transfer profile for resorbable implants demonstrating the load-sharing properties of the implant.
rounding cells), and mutagenicity (materials that cause genetic mutations within the cell). More specifically related to bone, the implant must also be osteocompatible, which means the material does not interfere with the normal bone healing process (7).
Although biocompatibility has a direct effect on how the tissue surrounding the device heals and is an important property of the implant, the main criteria related to implant function are the resorbability and mechanical properties. Once the device is implanted, it provides immediate mechanical stabilization to the site while the tissue heals. As the regenerating bone, ligaments, or tendons become stronger over time, the implant site becomes less dependent on the device and more dependent on the healing tissue. This concept is shown in Fig. 1. In this situation, the implant provides all of the mechanical support immediately following placement. As the device begins to degrade, the mechanical properties decrease over time and are gradually transferred to the new tissue. During this period, the regenerating tissue responds to the gradual loads and begins to remodel and become stronger. In the healing of musculoskeletal tissue, the sharing of the load between the implant and the tissue results in further regeneration. Once healing is complete, the load is fully transitioned to the tissue, which is now mechanically independent from the implant. Upon final resorption of the device, the site is left fully functional and entirely free of any implant material.
The ability to gradually transfer load to regenerating tissue is an important part of the musculoskeletal healing process. This characteristic is only found in resorbable materials. Although metallic implants offer effective load-bearing properties in applications such as joint replacement and certain spinal surgeries, these high strength materials do not resorb and do not effectively transfer loads to the implant site. Due to the high strength of metals, these implants bear the majority of the force at the site and can shield the surrounding tissues from any load. This phenomenon is called stress shielding and can actually cause bone to resorb in certain areas around the implant (8,9). The stress-shielding effect is based on a concept called Wolff ’s Law, which describes the ability of bone to

dynamically respond to the presence or lack of stress by changing its density and strength.
When bone is subjected to new loads, the additional stress stimulates bone formation and the tissue increases in strength and density. When the remodeling process is complete, the stronger tissue can now fully support the added load. However, when a high strength material such as metal is placed in bone, the bone surrounding the implant is shielded from the normal stresses, which results in a decrease in the strength and density of this tissue and possible bone resorption. This phenomenon can cause complications such as implant loosening or fracture of the implant site. Polymer and ceramic materials, on the other hand, have mechanical properties that are similar to bone, which allows them to share the stresses with newly regenerating tissue thereby preventing resorption and other stress-shielding complications (10–12).
Although load transfer and strength retention are common properties of all resorbable implants, not all surgical sites heal at the same rate. In fracture fixation applications where bone-to-bone contact is maintained, healing can be as short as 6–8 weeks. However, in applications such as spinal fusion where significant amounts of tissue need to be formed in the intervertebral space, the healing process can take up to 6–12 months. Based on the dependence of implant function on the surgical site, the material choice becomes an important part of implant development. The challenge in designing an implant lies in choosing a material that correctly matches the function and strength requirements of the surgical application, which can be accomplished through a thorough understanding of the function of the implant, the load requirements of the implant site, and the properties of the material.
RESORBABLE POLYMERS
One of the most versatile materials used in orthopedic surgery are polymers. Polymers are a group of materials that are produced through a chemical reaction that results in a long chain of repeating molecules called monomers. In addition to polymers composed of a single monomer repeating unit, there are other materials, called copolymers, that have two or more monomer repeating units. By combining different monomers, the properties of the resultant copolymer can be specifically modified to serve a certain purpose. This versatility can also be achieved by modifying the polymerization reaction and the postprocessing techniques used to create polymer implants. Table 1 shows a few
Table 1. Range of Common Properties Found in
Orthopedic Polymers
Property |
Range |
|
|
|
|
Resorbability |
Fully Resorbable |
Nonresorbable |
Strength |
Low Strength |
High Strength |
Moldability |
Flexible |
Rigid |
Physical State |
Gel/Liquid |
Solid |
Temperature |
Flexible at higher |
Rigid at all |
Sensitivity |
Temperature |
Temperatures |
Radiation Resistance |
Low |
High |
|
|
|
BIOMATERIALS, ABSORBABLE |
257 |
examples of the many properties that characterize polymers. These characteristics can be altered by changing the molecular weight, chemical structure, and morphology of the polymer or copolymer.
The molecular weight of a polymer is a measurement of the number of repeating units found in the entire molecule. During the formation of polymers and copolymers, the length of the molecule can be controlled to give a variety of molecular weights. The length of the polymer chain can be as small as a few thousand repeating units or as large as a million, which can have a significant effect on the degradation properties of the polymer. When a polymer breaks down, it occurs through random cleavage of the chemical bonds along the polymer chain. It is not until the polymer finally fragments into its monomer form that the material is absorbed by the surrounding tissue. Therefore, longer polymers chains with higher molecular weights will take a longer time to degrade because more bonds exist to the cleaved.
Additionally, the chemical structure can also affect degradation. As described previously, the backbone of a polymer consists of a long, continuous chain of monomer units linked together. In all resorbable polymers, it is the backbone of the polymer where degradation occurs. The typical linkage that allows polymers to break down is a carbon–oxygen–carbon (C-O-C) bond. This bond is found in ester, carbonate, carboxylic acid, and amide-based polymers. The degradation process occurs at this bond when the material is exposed to water. In a process called hydrolytic degradation, water molecules chemically react with the C-O-C bonds causing them to break apart at random areas throughout the polymer chain. The chemical structure of the polymer dictates the ability of the water molecules to access these bonds and start the degradation reaction. If the polymer is characterized by large bulky side chains or strong C-O-C bonds, it becomes difficult for the water molecule to penetrate the polymer chains to react with the backbone, which results in a prolonged degradation period. The opposite is true for polymers that tend to absorb water and do not have any large side chains. In these polymers, the water molecules can easily access the backbone and the degradation process proceeds at a relatively fast rate.
The final characteristic that can affect the degradation and strength of a polymer is the morphology. The morphology of the polymer refers to the orientation of the long polymer chains throughout the material. Polymer morphology can be classified into three groups: crystalline polymers, semicrystalline polymers, and amorphous polymers. The crystallinity of a polymer develops from areas within the material where the polymer chains are aligned and tightly packed together. This type of orientation forms dense crystalline regions within the random arrangement of the polymer chains. A highly organized polymer is considered crystalline, whereas a completely random orientation is considered amorphous. Semicrystalline polymers fall between these two extremes and exist with varying degrees of crystallinity (Fig. 2).
The effect of crystallinity on the degradation of the polymer is due to the tight orientation between the polymer chains in the crystalline regions. With highly crystalline
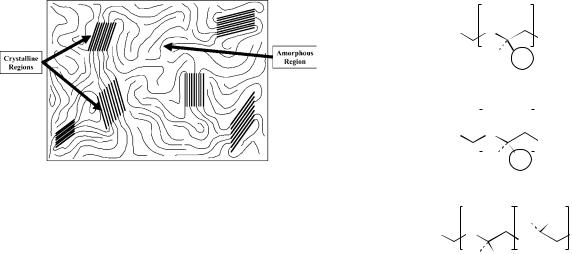
258 BIOMATERIALS, ABSORBABLE
Figure 2. Semicrystalline polymer showing orientation of amorphous regions and crystalline regions.
polymers, the degradation rate is very slow due to the difficulty of water gaining access to the C-O-C bonds. These polymers degrade at a rate much slower than polymers that are completely amorphous with no crystalline regions (13). The crystallinity also affects the mechanical properties of the polymer. The dense, organized areas within crystalline polymer make these regions stronger than the unorganized, amorphous regions. As a result, an increase in crystallinity translates into an increase in mechanical properties.
The ability to alter the properties of a polymer has resulted in thousands of different materials used in a wide range of applications. However, only a few of these polymers can be effectively used as medical implants due to the strict requirements of surgical implants. The following sections describe some of the polymers currently used in orthopedic surgery.
Poly(hydroxy acids)
Poly(hydroxy acids) were the first group of resorbable materials to be used in surgery (14). The main polymers in this family are poly(lactic acid) (PLA), poly(glycolic acid) (PGA), and the copolymer poly(lactide-co-glycolide) (PLG). The basic chemical structure of these materials in shown in Fig. 3. Originally, PLA and PGA were initially used as a degradable sutures (15–18). However, since their initial success in the wound closure field, both of these polymers have been fabricated into several orthopedic implants including screws (19,20), plates (19,21), pins (22–25), suture anchors (26), and bone grafting scaffolds (27–30). In addition, several new devices composed of the PLG copolymer have been developed over the past 10 years (31–35).
Although the chemical structure of PLA and PGA is somewhat similar, the presence of a methyl group (–CH3) in PLA significantly changes its physical properties compared with PGA. Comparatively, PGA has a lower strength and degrades in approximately 3–6 months, whereas certain forms of PLA can take 3–5 years to fully degrade. Although only a single methyl group differentiating PLA from PGA exists, the location of this side group close to the C-O-C bond makes it difficult for the water molecules to gain access to cleavage site, thereby prolonging degradation.
|
|
|
|
|
|
|
|
|
O |
|
|||||||
HO |
|
|
|
O |
|
|
|
nOH |
|
||||||||
|
|
|
|
|
|
|
|||||||||||
|
|
|
|
|
|
|
|
|
|
|
|
|
|||||
|
|
|
|
|
|
|
|
|
|
||||||||
|
|
|
O |
H |
CH3 |
|
|||||||||||
POLY (LACTIC ACID) |
|
||||||||||||||||
|
|
|
|
|
|
|
|
|
O |
|
|
|
|
|
|
||
|
|
|
|
|
|
|
|
|
|
|
|
|
|
|
|||
HO |
|
|
|
O |
|
|
|
nOH |
|
||||||||
|
|
|
|
|
|
|
|||||||||||
|
|
|
|
|
|
H |
|
|
|
|
|||||||
|
|
|
|
|
|
|
|
|
|||||||||
|
|
|
O |
H |
|
||||||||||||
POLY (GLYCOLIC ACID) |
|
||||||||||||||||
|
|
|
|
|
|
O |
H H |
|
|||||||||
HO |
O |
|
|
|
O |
|
|
|
|
OH |
|||||||
|
|
|
|
|
|
|
|||||||||||
|
|
|
|
H |
CH3 |
|
|
|
|
n |
|||||||
|
|
|
|
|
|
||||||||||||
|
|
|
|
|
|
|
|
|
|
|
|
||||||
O |
|
|
|
|
O |
|
POLY (LACTIDE-co-GLYCOLIDE)
Figure 3. Chemical structure of poly(lactic acid), poly(glycolic acid), and the copolymer poly(lactide-co-glycolide).
In addition, the methyl group in PLA also gives the polymer a unique chemical orientation. As a monomer, lactic acid is a molecule that can have two different molecular orientations: L-lactic acid and D-lactic acid. These isomers are based on the orientation of the methyl and hydrogen groups on the molecule. Figure 4 shows the chiral nature of the lactic acid molecule and the resulting stereoregular polymers: poly(L-lactic acid) (PLLA), poly (D-lactic acid (PDLA), and poly (D,L-lactic acid) (PDLLA). Although three forms of PLA exist, in the medical field, poly(L-lactic acid) is used more often than poly(D-lactic acid) because the degradation product is the same as naturally occurring L-lactic acid (13).
Using the various forms of PLA, polymers with significantly different properties can be synthesized. The effect of the starting isomer on the physical properties of the material is dramatically seen in the properties of PLLA and PDLLA. In Fig. 4, the chemical structure of poly (L-lactic acid) is represented by a long chain with all of the –CH3 groups on one side. This uniformity allows the chains to pack tightly together resulting in a highly crystalline material that has a high strength and long degradation period (3–5 years). Poly(D,L lactic acid), on the other hand, is characterized by either a random or alternating arrangement of the –CH3 groups and –H groups. This molecule orientation prevents the polymer chains from packing together, resulting in a completely amorphous polymer with a lower strength and shorter degradation profile (9–12 months). In addition, the polymerization of L-lactic acid and D,L-lactic acid together results in a copolymer with properties in between PLLA and PDLLA. In recent years, the 70:30 combination of poly(L/D,L lactic acid) has gained popularity in orthopedic applications due to its ability to retain its strength for 9– 12 months while being completely resorbed within 1.5–2 years (36–38). This copolymer appears to provide the best
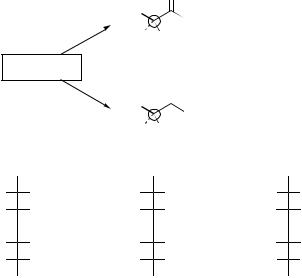
O
HO
OH
H CH3
Optically Active L-LACTIC ACID
Center
|
|
|
O |
|
|
||
|
|
HO |
|
|
OH |
|
|
|
|
|
|
|
|
||
|
|
|
|
|
|
|
|
|
|
CH3 |
H |
|
|
||
|
|
D-LACTIC ACID |
|
|
|||
H |
CH3 |
CH3 |
H |
H |
CH3 |
||
H |
CH3 |
CH3 |
H |
CH3 |
H |
||
H |
CH3 |
CH3 |
H |
H |
CH3 |
||
H |
CH3 |
CH3 |
H |
CH3 |
H |
||
H |
CH3 |
CH3 |
H |
H |
CH3 |
||
POLY(L-LACTIC ACID) |
POLY(D-LACTIC ACID) |
POLY(DL-LACTIC ACID) |
BIOMATERIALS, ABSORBABLE |
259 |
Figure 4. The optically active center in lactic acid is allows it to have two different molecular orientations. These orientations result in three types of stereoregular polymers.
of both worlds in that it has the strength retention of PLLA but has a degradation period only slightly longer than PDLLA.
In addition to the lactic acid-based copolymers, a combination of PLA and PGA has also been shown to be an effective implant material (31–35). Due to the large differences in the degradation properties of PLA and PGA, the poly(lactide-co-glycolide) (PLG) copolymer can be modified based on the PLA to PGA ratio to provide varying degradation periods. Common PLG copolymers used in orthopedic surgery have PLA/PGA ratios of 50:50, 75:25, and 85:15. This combination not only provides both slow and fast resorbing monomer units but also eliminates any crystallinity, making the copolymer completely amorphous. These materials have been commonly used as fracture implants due to the shorter 6–12 month degradation period.
Although PLA and PGA polymers have been successfully used in patients for several years, there have been certain cases where the abundance of acidic monomers at the site has caused inflammation and bone resorption (39– 48). When PLA and PGA polymers near the end of degradation, they release lactic acid and glycolic acid, respectively. Although these degradation products can be metabolized by the body, if the surrounding tissue cannot absorb the acid in a timely manner, the build up of acid and resultant drop in pH at the implant site can cause bone to resorb. Historically, this effect has mainly been seen in the fast-resorbing PGA implants; however, a few cases have been reported with PLA (43,46,49,50). Although the bone resorption complication is detrimental to the healing of the implant site, the complication rate has been relatively low. In a review of over 2000 patients by Bostman, only 5% of the patients have shown implantassociated reactions (44).
Additionally, the copolymers PLG and PLDLLA have been shown to possess a more osteocompatible degradation profile due to a gradual release of the acidic byproducts (36,51–56), which has minimized acid dumping and the
associated bone resorption complications. In a study by Eppley et al. (35), 1883 patients treated with PLG plates and screws for bone fixation in craniofacial procedures showed an implant-related complication rate of only 0.5%, which was well below the 5% rate reported by Bostman for PGA and PLA implants. Overall, the PLG and PLDLLA copolymers have been shown to be effective devices for fracture fixation, bone graft containment, and soft tissue fixation, and have begun to replace the outdated PLA and PGA devices (37,38,57,58).
Polycarbonates
Another group of resorbable polymers are the polycarbonates. Although the majority of the polymers and copolymers within the polycarbonate family are nonresorbable plastics used for industrial applications, a select few exist that are resorbable and can be used as orthopedic implants. One group of medical-grade polycarbonates are the copolymers based off of poly(trimethylene-carbonate) (PTMC) and poly(glycolic acid) or poly(lactic acid). These combinations offer the combined advantage of the processing versatility of PTMC and the resorbability and strength of PLA and PGA. The PTMC copolymers have been used for soft tissue fixation in shoulder surgery as suture anchors and soft tissue tacks (59–61).
Although the PTMC copolymers with PGA and PLA offer improved implant properties compared with PTMC alone, the degradation of the material still produces acidic monomers. In order to avoid the issues with glycolic acidand lactic acid-based polymers and copolymers, an amino acid-based polycarbonate was developed by Joachim Kohn at Rutgers University. Designed specifically for orthopedic applications, the amino acid poly(carbonates) combine the biocompatibility of individual amino acids with the strength and processability of standard industrial poly(carbonates) (62–64). One such promising polymer, poly(DTE carbonate), is derived from the amino acid
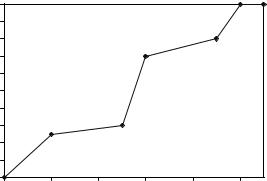
260 BIOMATERIALS, ABSORBABLE
tyrosine and has been shown to have excellent strengthretention properties, an optimal degradation profile, and biocompatible degradation products (65–68). Based on large amount of characterization data, a material safety file has been recently established at the U.S. Food and Drug Administration (FDA) that allows manufacturers to begin development of poly(DTE carbonate) implants. Due to the advantages of poly(DTE carbonate) over conventional resorbable polymers, amino acid-based poly(carbonate) implants may soon be a common sight in orthopedic operating rooms.
Other Resorbable Polymers
In addition to the widely used PLA and PGA polymers and the up-and-coming amino acid-based poly(carbonates), several other polymers have applications as medical devices. Although not specifically used in orthopedics, the poly(anhydride) family of polymers developed by Robert Langer at MIT has been effectively used as drugdelivery vehicles (69–73). The function of these resorbable implants is to provide a sustained and controlled release of drugs to a specific implant site. The device functions by releasing molecules entrapped within the implant as it degrades. Another polymer, poly(dioxanone), has been used as a resorbable suture material for several years (74–80). The flexibility of this polymer enables it to be used as a monofilament suture instead of the typical braided fiber of PGA, which provides the suture with an improved ability to move through tissue with less friction, thereby minimizing the tearing and pulling of the surrounding areas (81,82). Looking specifically at orthopedic applications, additional polymers currently in development include poly(caprolactone) (83–86), poly- (hydroxybutyrate) (87–89), polyurethanes (90–93), and poly(phosphazenes) (94–96).
RESORBABLE CALCIUM CERAMICS
Aside from the polymers, the other group of resorbable implant materials are the calcium-based ceramics. Due to the similarity of these materials with the mineral content of bone, hydroxyapatite [Ca10(PO4)6(OH)2], calcium ceramics are highly biocompatible and osteocompatible materials that have a long history of clinical use. These materials are typically used in orthopedic surgery to fill voids in bone as self-setting cements or as porous blocks and granules.
Calcium Sulfate
One the first materials to ever be used as a filler for bone defects was calcium sulfate (Plaster of Paris) (97). In its dehydrated form (calcium sulfate hemihydrate), this material undergoes a chemical reaction when mixed with water that allows it to function as a resorbable cement. As the cement reacts, it transforms from a slurry, to a paste, to a dough, and then fully sets into its final hardened form (calcium sulfate dihydrate). This reaction is exothermic in that it produces heat; however, the increase in temperature is only slightly above body temp (37 8C). Figure 5 shows a
|
100% |
|
|
|
|
Solid |
|
|
|
|
|
|
|
|
90% |
|
|
|
|
|
|
80% |
|
|
|
Putty |
|
|
|
|
|
|
|
|
reacted |
70% |
|
|
|
|
|
60% |
|
|
|
|
|
|
50% |
|
|
|
|
|
|
Percent |
|
|
|
|
|
|
40% |
|
Paste |
|
|
|
|
30% |
|
|
|
|
||
Slurry |
|
|
|
|
||
|
20% |
|
|
|
|
|
|
|
|
|
|
|
|
|
10% |
|
|
|
|
|
|
0% |
|
|
|
|
|
|
0 |
2 |
4 |
6 |
8 |
10 |
Time (min)
Figure 5. Typical setting reaction and phase changes for a calcium sulfate cement.
typical timeline of the calcium sulfate setting reaction. In the slurry and paste form, the calcium sulfate is able to be added to a syringe and injected to the bone graft site. Near the end of the reaction, the cement becomes much thicker and has a putty-like consistency. During this phase, the doughy cement can be molded into a variety of shapes and provides a custom fit when placed directly at the implant site. Once the cement has fully hardened, it can be shaped by using powered surgical instruments such as osteotomes, burrs, and drills.
The resorption of calcium sulfate graft materials is based on the microstructure of the fully hardened cement. Figure 6 shows electron micrographs of the surface of fully reacted calcium sulfate dihydrate. These high magnification images show small calcium sulfate crystals packed together in a microporous structure. Upon implantation, the presence of these small pores allows the calcium sulfate to absorb water throughout the cement. Unlike polymers, which undergo active breakdown of the polymer chains, calcium sulfate materials are slowly dissolved by the water. As the material dissolves, Ca2þ and SO4 3 ions are released over a 6–8 week period. During healing, bone formation initially begins on the outer area of the calcium sulfate and progresses inward as the cement slowly breaks apart. During the resorption process, the dissolution of the calcium sulfate material aids bone formation by providing a direct source Ca2þ ions to the surrounding osteoblasts. These cells absorb the calcium and use it during the mineralization phase of bone regeneration. From a mechanical standpoint, the hardened cement can provide initial stabilization to the site, but quickly loses it strength as the calcium sulfate begins to fragment. Although the strength of the calcium sulfate quickly decreases within the first few weeks, additional bone regeneration takes place within the cement and the implant site becomes mechanically stable. At the 6–8 week period, the majority of the calcium sulfate is resorbed by the body and has been replaced by bone.
In general, calcium sulfate cements and implants offer an effective means to fill small voids in bone resulting from cysts, tumors, or fractures (98–101). The initial strength
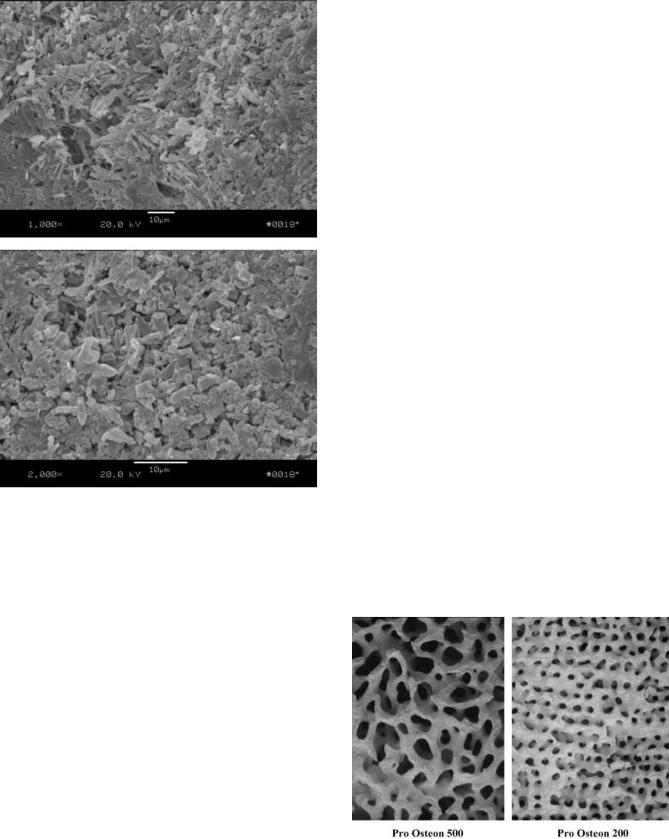
Figure 6. High magnification scanning electron micrographs of fully reacted calcium sulfate dihydrate showing crystalline structure and microporosity (1000X and 2000X magnification).
can also help maintain the spacing of fracture fragments and aid in placement of additional hardware. The moldability of the cement allows a custom fit to the defect site and makes the material easy to use. However, due to the quick resorption time and quick loss in strength, this material can not be effectively used in large defects or in areas under high mechanical loads. In these applications, supplemental hardware and grafting materials are needed to ensure complete bone regeneration (102,103). From a commercial standpoint, calcium sulfate graft materials are available in a cement form (requires mixing at the time of surgery) or in a preformed pellet form (fully reacted calcium sulfate dihydrate).
Calcium Phosphate. Calcium phosphates are another class of calcium containing bone graft materials that offer different properties than the calcium sulfates. As the name describes, these material are composed of varying amounts of calcium (Ca2þ) and phosphate (PO4 3). One of the first calcium phosphate materials to be used as a bone graft was hydroxyapatite, which was chosen because it is the main inorganic component of bone accounting for 40% of its
BIOMATERIALS, ABSORBABLE |
261 |
weight. Most calcium phosphate graft materials are produced synthetically and can be chemically altered to create materials with different properties. By slightly varying the calcium-to-phosphate ratio, the resorption times and mechanical properties of these materials can be significantly altered. Hydroxyapatite [Ca10(PO4)6(OH)2] with a Ca/P ratio of 1.67 has slow resorption rate, which, depending on crystallinity, can be as little are 2–5% resorption per year. Tricalcium phosphate Ca3(PO4)2 has a ratio of 1.5, which results in a much faster resorption time of 9–12 months.
Due to the chemical composition of calcium phosphates, the mechanism of resorption is different than the dissolution mechanism seen with calcium sulfates. The chemical similarity of calcium phosphates to bone results in a cell-mediated resorption profile. During healing, boneresorbing cells called osteoclasts migrate to the surface of the calcium phosphate ceramics. Once activated, the osteoclasts release specific enzymes that dissolve the calcium phosphate into its base ions. As the osteoclasts tunnel through the calcium phosphate, bone-forming cells called osteoblasts trail behind filling in the region with new tissue. Similar to calcium sulfate, the calcium ions resulting from the resorption process are transported to the osteoblasts, which create new mineralized bone. Over time, the entire structure is slowly dissolved by the osteoclasts and replaced with new bone.
To facilitate this type of resorption process, many of the calcium phosphate bone graft materials exist as porous scaffolds (104–109). A typical example of an osteoconductive calcium phosphate bone graft scaffold is shown in Fig. 7. This material, called Pro Osteon (developed and manufactured by Interpore Cross), was one of the first porous calcium phosphates used in orthopedics (110–113). Derived from sea coral, it is fabricated by chemically converting the calcium carbonate skeleton of the coral into hydroxyapatite. This reaction can be run to completion to give a implant composed entirely of hydroxyapatite or intentionally stopped to result in an implant with a thin (4–10 mm) surface of hydroxyapatite over the calcium carbonate skeleton. The conversion of coral to Pro Osteon
Figure 7. Photographs of a two commercially available calcium phosphate scaffolds derived from coral (Interpore Cross, Irvine, CA).

262 BIOMATERIALS, ABSORBABLE
allows the relatively short degradation time of calcium carbonate (6–8 weeks) to be prolonged to 8–18 months for Pro Osteon R (HA layer on the calcium carbonate skeleton) and to 3–5 years for Pro Osteon HA (fully converted hydroxyapatite). With a natural pore structure similar to cancellous bone, the Pro Osteon graft materials offer an effective scaffold for new bone growth. Since development of the Pro Osteon bone graft materials, several other porous calcium phosphates have entered the market. These materials are synthetically made to mimic the porosity of cancellous bone, which is done through various foaming and void creation techniques.
In contrast to calcium sulfate graft materials, the slower resorption profile of porous calcium phosphate ceramics allow these material to be used in larger defects. In this scenario, the graft serves as a cellular ‘‘bridge’’ for continued bone growth. In bone grafting surgery, once a defect reaches a size when it can no longer completely heal itself, it is called a critical-sized defect. Typical bone regeneration can bridge empty gaps of up to 4 mm, but anything larger will not fill in with bone. A porous ceramic scaffold alleviates this problem by providing the means for bone to grow across the entire defect.
This effect was demonstrated in a study by Holmes who implanted a block of Pro Osteon 500R (calcium carbonate scaffold with an HA coating) into a rabbit tibial defect (114). The healing sequence of the this scaffold is shown in Fig. 8. As seen from cross sectional image of the implant before implantation (Fig. 8a), the structure is characterized by an open pore structure (black regions) within areas of calcium carbonate/HA ceramic (light-gray regions). After initial placement of the porous ceramic, cells migrated to the graft site and began to infiltrate the pore system. At the same time, proteins were released from surrounding bone and blood cells to stimulate the bone regeneration process, which was seen in the 6 week histology of the Pro Osteon 500R implant (Fig. 8b). In this
Figure 8. Typical healing mechanism of a porous ceramic implanted into a rabbit tibial defect. (Figure A – 0 weeks; Figure B – 6 weeks; Figure C – 12 weeks; Figure D – 24 weeks).
image, bone formation was evident within the porosity of the scaffold, and osteoclasts were seen resorbing the scaffold (arrows). By 12 weeks, further bone growth was seen within the porosity, and significant portions of the scaffold were replaced by bone. At the 24 week time point, the scaffold was fully replaced by bone with the exception of the thin HA layer that once covered the calcium carbonate. As seen from this study, porous ceramics are capable of functioning as a scaffold for bone growth. The pore system allowed for immediate bone regeneration and the resorbability allowed the implant to be completely replaced by bone.
In addition to porous blocks and granules, calcium phosphates are also used in cement form (115–119). In this application, the base components that create calcium phosphates are provided in an unreacted form. With the addition of water, dilute acid, or other initiators, a chemical reaction takes place, and the components are converted to calcium phosphate. The result is a moldable paste or putty that can be shaped to the graft site and hardens into a solid mass. Although these cements have longer resorption times than calcium sulfate cements and can be used in broader applications, the resulting hardened cement does not possess the porosity to function as a scaffold for bone repair, which has limited the use of calcium phosphate cerments because surgeons prefer the porous blocks and granules over the self-setting cements.
RESORBABLE COMPOSITES
As discussed, both polymers and ceramics have properties suitable for fabricating orthopedic implants. However, certain drawbacks exist with these materials that can not be avoided no matter how the material is fabricated or chemically altered. One technique for combining the desirable properties of two or more materials is the fabrication of a composite. Composites used in the medical device area are fabricated by physically mixing two or more resorbable materials. One of the most common composite combinations is the creation of a polymer-ceramic composite. On their own, ceramics are excellent substrates for new bone growth due to the chemical similarity with bone mineral. However, their brittleness limits their use in load-bearing applications. Polymers, on the other hand, are elastomeric materials that can flex under deformation without major structural collapse. The combination of these two materials results in a high strength, yet ductile composite that allows for direct bone attachment on its surface. In this combination, the polymer adds to the overall mechanical properties of the composite, whereas the ceramic allows for bone formation directly on the ceramic phase.
The fabrication of a composite is a relatively straightforward process. Typically, ceramic particles in the shape of spheres or fibers are added to the polymer during processing. The various orientations of the particles within a polymer are shown in Fig. 9. As seen from these illustrations, each particle is surrounded by the polymer and serves to reinforce the polymer phase and improve it mechanical properties. Once fabricated in a block or rod
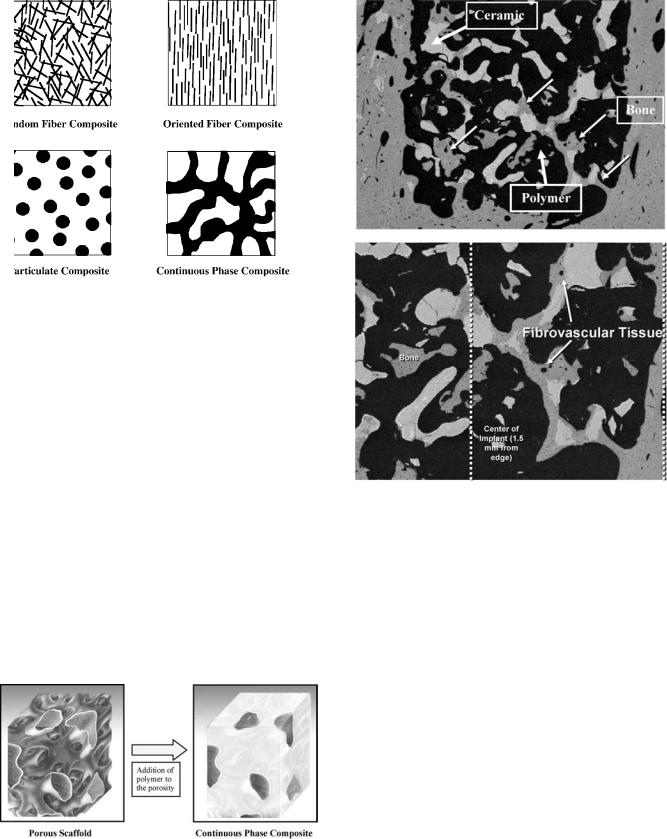
Figure 9. Orientation of various types of polymer-ceramic composites (ceramic is depicted as the black particles).
form, composites of these different types can be machined into a variety of implants such as fracture screws, pins, and plates. During the machining process, the ceramic on the outer surfaces of the implant are exposed. From a bone implant standpoint, the presence of the exposed calcium ceramic particles on the surface of the polymer aids in creating a solid bone-to-implant interface. In comparison, pure polymer implants typically heal with limited bone contact or a continuous layer of fibrous tissue usually covering the surface. Although the implant can still provide stabilization, it is not directly bonded to the surrounding bone. A composite implant improves on the stabilizing effect of the device through this bone-bonding ability.
In addition to the particulate ceramic composites, a new type of composite has recently been developed by Interpore Cross (Irvine, CA). This novel material consists of two intact, continuous phases of polymer and ceramic. Shown in Fig. 10, a continuous phase composite (CPC) is the result of infiltrating a porous ceramic block with polymer. The
Figure 10. A continuous phase composite is formed when a polymer is infiltrated into the porosity a porous, ceramic scaffold. The result is a solid block with an intact polymer and ceramic phase.
BIOMATERIALS, ABSORBABLE |
263 |
Figure 11. Backscattered electron microscope images demonstrating bone and blood vessel in-growth into a CPC implant (bone is shown in gray, ceramic is white, and polymer is black).
end result is a composite material with continuous seams of ceramic running through the polymer. Similar to the particulate composites, the CPC material will allow for bone growth on the surface and into the ceramic regions. However, the continuity of the ceramic phase throughout the composite gives the material a unique ability to allow for bone to penetrate into the center of a CPC implant. Figure 11 shows the histology a CPC implant composed of the Pro Osteon porous ceramic infiltrated with poly(L/D,L lactic acid) implanted in a sheep femur at 9 months. This backscattered electron microscope image shows the ability of a CPC implant to support bone and blood vessel ingrowth into the center of the implant wall. In addition to acting as a structural implant, a CPC device also functions as an eventual scaffold for bone in-growth. From a healing standpoint, this type of composite will result in more bone formation at the site. Additionally, the presence of bone and blood vessels within the implant wall significantly improves the ability of the tissue to absorb the degradation products. Typically occurring at the surface of polymer implants, the presence of bone within the CPC material allows resorption throughout the entire device. This new
264 BIOMATERIALS, ABSORBABLE
composite is currently being investigated for use as spinal fusion implants, fracture screws and plates, interference screws, and suture anchors.
CONCLUSION
As seen from this article, resorbable polymers and ceramics possess the desired properties needed for orthopedic implants. They have been shown to be versatile materials with a range in degradation rates and mechanical properties. The resorbable nature of these devices allows them to provide temporary stabilization and mechanical support. Combined with the ability to be completely resorbed by the body and replaced by natural tissue, these implants are highly desirable alternatives to their nonresorbing counterparts. The elimination of long-term implant complications and the ability to share load with regenerating tissues are large driving forces behind the use of these implants in orthopedics. With further advancements in biomaterial research, resorbable implants may soon become the standard of care.
BIBLIOGRAPHY
Cited References
1.History of Total Joint Replacement. Utah Hip and Knee Center. Available: http://www.utahhipandknee.com/history. htm. Accessed September 24, 2004.
2.History of Innovation. Zimmer Corporate Website. Available:http://www.zimmer.com/ctl?op=global&action=1&id= 1420&template= CP. Accessed September 24, 2004.
3.Parker DA, Dunbar MJ, Rorabeck CH. Extensor mechanism failure associated with total knee arthroplasty: Prevention and management. J Am Acad Orthop Surg 2003;11:238–247.
4.Ries MD. Complications in primary total hip arthroplasty: Avoidance and management: wear. Instrum Course Lect 2003;52:257–265.
5.Sochart DH. Relationship of acetabular wear to osteolysis and loosening in total hip arthroplasty. Clin Orthop 1999; 135–150.
6.Moreland JR. Mechanisms of failure in total knee arthroplasty. Clin Orthop 1988; 49–64.
7.Borden MD. The development of bone graft materials using various formulations of demineralized bone matrix. In Laurencin CT, editor, Bone Graft Substitutes. Conshohocken, (PA): ASTM International; 2003.
8.Uhthoff HK, Finnegan M. The effects of metal plates on posttraumatic remodelling and bone mass. J Bone Joint Surg Br 1983;65:66–71.
9.van der EM, Dijkema AR, Klein CP, Patka P, Haarman HJ. Tissue reaction on PLLA versus stainless steel interlocking nails for fracture fixation: An animal study. Biomaterials 1995;16:103–106.
10.Bos RR, Rozema FR, Boering G, Nijenhuis AJ, Pennings AJ, Verwey AB. Bio-absorbable plates and screws for internal fixation of mandibular fractures. A study in six dogs. Int J Oral Maxillofac Surg 1989;18:365–369.
11.Viljanen J, Kinnunen J, Bondestam S, Majola A, Rokkanen P, Tormala P. Bone changes after experimental osteotomies fixed with absorbable self-reinforced poly-L-lactide screws or metallic screws studied by plain radiographs, quantitative computed tomography and magnetic resonance imaging. Biomaterials 1995;16:1353–1358.
12.van der EM, Klein CP, Blieck-Hogervorst JM, Patka P, Haarman HJ. Bone tissue response to biodegradable polymers used for intra medullary fracture fixation: A long-term in vivo study in sheep femora. Biomaterials 1999; 20:121–128.
13.Engelberg I, Kohn J. Physico-mechanical properties of degradable polymers used in medical applications: A comparative study. Biomaterials 1991;12:292–304.
14.Kulkarni RK, Pani KC, Neuman C, Leonard F. Polylactic acid for surgical implants. Arch Surg 1966;93:839–843.
15.Chu CC. A comparison of the effect of pH on the biodegradation of two synthetic absorbable sutures. Ann Surg 1982;195:55–59.
16.Chu CC. Mechanical properties of suture materials: An important characterization. Ann Surg 1981;193:365–371.
17.Chu CC, Campbell ND. Scanning electron microscopic study of the hydrolytic degradation of poly(glycolic acid) suture. J Biomed Mater Res 1982;16:417–430.
18.Chu CC. The in-vitro degradation of poly(glycolic acid) sutures–effect of pH. J Biomed Mater Res 1981;15:795– 804.
19.Leenslag JW, Pennings AJ, Bos RR, Rozema FR, Boering G.
Resorbable materials of poly(L-lactide). VI. Plates and screws for internal fracture fixation. Biomaterials 1987;8:70– 73.
20.Tuompo P, Partio EK, Jukkala-Partio K, Pohjonen T, Helevirta P, Rokkanen P. Comparison of polylactide screw and expansion bolt in bioabsorbable fixation with patellar tendon bone graft for anterior cruciate ligament rupture of the knee. A preliminary study. Knee Surg Sports Traumatol Arthrosc 1999;7:296–302.
21.Koskikare K, Hirvensalo E, Patiala H, Rokkanen P, Pohjonen T, Tormala P, Lob G. Fixation of osteotomies of the distal femur with absorbable, self-reinforced, poly-L-lactide plates. An experimental study on rabbits. Arch Orthop Trauma Surg 1997;116:352–356.
22.Makela EA, Vainionpaa S, Vihtonen K, Mero M, Laiho J, Tormala P, Rokkanen P. Healing of physeal fracture after fixation with biodegradable self-reinforced polyglycolic acid pins. An experimental study on growing rabbits. Clin Mater 1990;5:1–12.
23.Pihlajamaki H, Bostman O, Hirvensalo E, Tormala P, Rokkanen P. Absorbable pins of self-reinforced poly-L-lactic acid for fixation of fractures and osteotomies. J Bone Joint Surg Br 1992;74:853–857.
24.Tormala P, Vasenius J, Vainionpaa S, Laiho J, Pohjonen T, Rokkanen P. Ultra-high-strength absorbable self-reinforced polyglycolide (SR-PGA) composite rods for internal fixation of bone fractures: In vitro and in vivo study. J Biomed Mater Res 1991;25:1–22.
25.Vainionpaa S, Kilpikari J, Laiho J, Helevirta P, Rokkanen P, Tormala P. Strength and strength retention in vitro, of absorbable, self-reinforced polyglycolide (PGA) rods for fracture fixation. Biomaterials 1987;8:46–48.
26.Barber FA, Deck MA. The in vivo histology of an absorbable suture anchor: A preliminary report. Arthroscopy 1995;11:77–81.
27.Borden M, El Amin SF, Attawia M, Laurencin CT. Structural and human cellular assessment of a novel microsphere-based tissue engineered scaffold for bone repair. Biomaterials 2003;24:597–609.
28.Borden M, Attawia M, Laurencin CT. The sintered microsphere matrix for bone tissue engineering: in vitro osteoconductivity studies. J Biomed Mater Res 2002;61:421–429.
29.Borden M, Attawia M, Khan Y, Laurencin CT. Tissue engineered microsphere-based matrices for bone repair: design and evaluation. Biomaterials 2002;23:551–559.
30.Thomson RC, Yaszemski MJ, Powers JM, Mikos AG. Fabrication of biodegradable polymer scaffolds to engineer trabecular bone. J Biomater Sci Polym Ed 1995;7:23–38.
31.Hollier LH, Rogers N, Berzin E, Stal S. Resorbable mesh in the treatment of orbital floor fractures. J Craniofac Surg 2001;12:242–246.
32.Edwards RC, Kiely KD, Eppley BL. The fate of resorbable poly-L-lactic/polyglycolic acid (LactoSorb) bone fixation devices in orthognathic surgery. J Oral Maxillofac Surg 2001;59:19–25.
33.Edwards RC, Kiely KD, Eppley BL. Resorbable PLLA-PGA screw fixation of mandibular sagittal split osteotomies. J Craniofac Surg 1999;10:230–236.
34.Westermark A. LactoSorb resorbable osteosynthesis after sagittal split osteotomy of the mandible: A 2-year followup. J Craniofac Surg 1999;10:519–522.
35.Eppley BL, Morales L, Wood R, Pensler J, Goldstein J, Havlik RJ, Habal M, Losken A, Williams JK, Burstein F, Rozzelle AA, Sadove AM. Resorbable PLLA-PGA plate and screw fixation in pediatric craniofacial surgery: Clinical experience in 1883 patients. Plast Reconstr Surg 2004;114:850–856.
36.Toth JM, Wang M, Scifert JL, Cornwall GB, Estes BT, Seim HB, III, Turner AS. Evaluation of 70/30 D,L-PLa for use as a resorbable interbody fusion cage. Orthopedics 2002;25: s1131–s1140.
37.Vaccaro AR, Madigan L. Spinal applications of bioabsorbable implants. Orthopedics 2002;25:s1115–s1120.
38.Lowe TG, Coe JD. Bioresorbable polymer implants in the unilateral transforaminal lumbar interbody fusion procedure. Orthopedics 2002;25:s1179–s1183.
39.Bostman OM. Osteolytic changes accompanying degradation of absorbable fracture fixation implants. J Bone Joint Surg Br 1991;73:679–682.
40.Pelto-Vasenius K, Hirvensalo E, Vasenius J, Rokkanen P. Osteolytic changes after polyglycolide pin fixation in chevron osteotomy. Foot Ankle Int 1997;18:21–25.
41.Bostman OM. Reaction to biodegradable implants. J Bone Joint Surg Br 1993;75:336–337.
42.Bostman OM. Intense granulomatous inflammatory lesions associated with absorbable internal fixation devices made of polyglycolide in ankle fractures. Clin Orthop 1992; 193– 199.
43.Bostman OM. Osteoarthritis of the ankle after foreign-body reaction to absorbable pins and screws: A threeto nine-year follow-up study. J Bone Joint Surg Br 1998;80:333–338.
44.Bostman OM, Pihlajamaki HK. Adverse tissue reactions to bioabsorbable fixation devices. Clin Orthop 2000; 216–227.
45.Bostman O, Pihlajamaki H. Clinical biocompatibility of biodegradable orthopedic implants for internal fixation: A review. Biomaterials 2000;21:2615–2621.
46.Rovinsky D, Nissen TP, Otsuka NY. Osteolytic reaction to polylevolactic acid fracture fixation. Orthopedics 2001;24: 177–179.
47.Bostman O, Hirvensalo E, Makinen J, Rokkanen P. Foreignbody reactions to fracture fixation implants of biodegradable synthetic polymers. J Bone Joint Surg Br 1990;72:592– 596.
48.Taylor MS, Daniels AU, Andriano KP, Heller J. Six bioabsorbable polymers: in vitro acute toxicity of accumulated
degradation products. J Appl Biomater 1994;5:151– 157.
49.Bergsma JE, de Bruijn WC, Rozema FR, Bos RR, Boering G. Late degradation tissue response to poly(L-lactide) bone plates and screws. Biomaterials 1995;16:25–31.
50.Bergsma EJ, Rozema FR, Bos RR, de Bruijn WC. Foreign body reactions to resorbable poly(L-lactide) bone plates and
BIOMATERIALS, ABSORBABLE |
265 |
screws used for the fixation of unstable zygomatic fractures. J Oral Maxillofac Surg 1993;51:666–670.
51.Lanman TH, Hopkins TJ. Lumbar interbody fusion after treatment with recombinant human bone morphogenetic protein-2 added to poly(L-lactide-co-D,L-lactide) bioresorbable implants. Neurosurg Focus 2004;16:E9.
52.Couture DE, Branch CL, Jr. Posterior lumbar interbody fusion with bioabsorbable spacers and local autograft in a series of 27 patients. Neurosurg Focus 2004;16:E8.
53.Vaccaro AR, Robbins MM, Madigan L, Albert TJ, Smith W, Hilibrand AS. Early findings in a pilot study of anterior cervical fusion in which bioabsorbable interbody spacers were used in the treatment of cervical degenerative disease. Neurosurg Focus 2004;16:E7.
54.Cornwall GB, Ames CP, Crawford NR, Chamberlain RH, Rubino AM, Seim HB, III, Turner AS. In vivo evaluation of bioresorbable polylactide implants for cervical graft containment in an ovine spinal fusion model. Neurosurg Focus 2004;16:E5.
55.Lippman CR, Hajjar M, Abshire B, Martin G, Engelman RW, Cahill DW. Cervical spine fusion with bioabsorbable cages. Neurosurg Focus 2004;16:E4.
56.Robbins MM, Vaccaro AR, Madigan L. The use of bioabsorbable implants in spine surgery. Neurosurg Focus 2004;16:E1.
57.Ames CP, Crawford NR, Chamberlain RH, Cornwall GB, Nottmeier E, Sonntag VK. Feasibility of a resorbable anterior cervical graft containment plate. Orthopedics 2002;25: s1149–s1155.
58.DiAngelo DJ, Kitchel S, McVay BJ, Scifert JL, Cornwall GB. Bioabsorbable anterior lumbar plate fixation in conjunction with anterior interbody fusion cages. Orthopedics 2002;25: s1157–s1165.
59.Speer KP, Warren RF, Pagnani M, Warner JJ. An arthroscopic technique for anterior stabilization of the shoulder with a bioabsorbable tack. J Bone Joint Surg Am 1996;78:1801–1807.
60.Warner JJ, Miller MD, Marks P, Fu FH. Arthroscopic Bankart repair with the Suretac device. Part I: Clinical observations. Arthroscopy 1995;11:2–13.
61.Warner JJ, Miller MD, Marks P. Arthroscopic Bankart repair with the Suretac device. Part II: Experimental observations. Arthroscopy 1995;11:14–20.
62.Bourke SL, Kohn J. Polymers derived from the amino acid L-tyrosine: polycarbonates, polyarylates and copolymers with poly(ethylene glycol). Adv Drug Deliv Rev 2003;55:447– 466.
63.Ertel SI, Kohn J. Evaluation of a series of tyrosine-derived polycarbonates as degradable biomaterials. J Biomed Mater Res 1994;28:919–930.
64.Ertel SI, Kohn J, Zimmerman MC, Parsons JR. Evaluation of poly(DTH carbonate), a tyrosine-derived degradable polymer, for orthopedic applications. J Biomed Mater Res 1995;29:1337–1348.
65.Choueka J, Charvet JL, Koval KJ, Alexander H, James KS, Hooper KA, Kohn J. Canine bone response to tyrosinederived polycarbonates and poly(L-lactic acid). J Biomed Mater Res 1996;31:35–41.
66.Tangpasuthadol V, Pendharkar SM, Peterson RC, Kohn J. Hydrolytic degradation of tyrosine-derived polycarbonates, a class of new biomaterials. Part II: 3-yr study of polymeric devices. Biomaterials 2000;21:2379–2387.
67.Chaikof EL, Matthew H, Kohn J, Mikos AG, Prestwich GD, Yip CM. Biomaterials and scaffolds in reparative medicine. Ann N Y Acad Sci 2002;961:96–105.
68.Kohn J, Langer R. Poly(iminocarbonates) as potential biomaterials. Biomaterials 1986;7:176–182.
266BIOMATERIALS, ABSORBABLE
69.Ibim SM, Uhrich KE, Bronson R, El Amin SF, Langer RS, Laurencin CT. Poly(anhydride-co-imides): in vivo biocompatibility in a rat model. Biomaterials 1998;19:941–951.
70.Ibim SE, Uhrich KE, Attawia M, Shastri VR, El Amin SF, Bronson R, Langer R, Laurencin CT. Preliminary in vivo report on the osteocompatibility of poly(anhydride-co-imides) evaluated in a tibial model. J Biomed Mater Res 1998;43:374– 379.
71.Uhrich KE, Ibim SE, Larrier DR, Langer R, Laurencin CT. Chemical changes during in vivo degradation of poly(anhydride-imide) matrices. Biomaterials 1998;19: 2045–2050.
72.Katti DS, Lakshmi S, Langer R, Laurencin CT. Toxicity, biodegradation and elimination of polyanhydrides. Adv Drug Deliv Rev 2002;54:933–961.
73.Attawia MA, Uhrich KE, Botchwey E, Langer R, Laurencin CT. In vitro bone biocompatibility of poly (anhydride- co-imides) containing pyromellitylimidoalanine. J Orthop Res 1996;14:445–454.
74.Ray JA, Doddi N, Regula D, Williams JA, Melveger A. Polydioxanone (PDS), a novel monofilament synthetic absorbable suture. Surg Gynecol Obstet 1981;153:497–507.
75.Ping OC, Cameron RE. The hydrolytic degradation of polydioxanone (PDSII) sutures. Part I: Morphological aspects. J Biomed Mater Res 2002;63:280–290.
76.Ping OC, Cameron RE. The hydrolytic degradation of polydioxanone (PDSII) sutures. Part II: Micromechanisms of deformation. J Biomed Mater Res 2002;63:291–298.
77.Ray JA, Doddi N, Regula D, Williams JA, Melveger A. Polydioxanone (PDS), a novel monofilament synthetic absorbable suture. Surg Gynecol Obstet 1981;153:497–507.
78.Bartholomew RS. PDS (polydioxanone suture): A new synthetic absorbable suture in cataract surgery. A preliminary study. Ophthalmologica 1981;183:81–85.
79.Lerwick E. Studies on the efficacy and safety of polydioxanone monofilament absorbable suture. Surg Gynecol Obstet 1983;156:51–55.
80.Cohen EL, Kirschenbaum A, Glenn JF. Preclinical evaluation of PDS (polydioxanone) synthetic absorbable suture vs chromic surgical gut in urologic surgery. Urology 1987;30:369– 372.
81.Apt L, Henrick A. ‘‘Tissue-drag’’ with polyglycolic acid (Dexon) and polyglactin 910 (Vicryl) sutures in strabismus surgery. J Pediatr Ophthalmol 1976;13:360–364.
82.Homsy CA, McDonald KE, Akers WW, Short C, Freeman BS. Surgical suture-canine tissue interaction for six common suture types. J Biomed Mater Res 1968;2:215–230.
83.Rhee SH, Lee YK, Lim BS, Yoo JJ, Kim HJ. Evaluation of a novel poly(epsilon-caprolactone)-organosiloxane hybrid material for the potential application as a bioactive and degradable bone substitute. Biomacromolecules 2004;5: 1575–1579.
84.Rhee SH. Bone-like apatite-forming ability and mechanical properties of poly(epsilon-caprolactone)/silica hybrid as a function of poly(epsilon-caprolactone) content. Biomaterials 2004;25:1167–1175.
85.Ciapetti G, Ambrosio L, Savarino L, Granchi D, Cenni E, Baldini N, Pagani S, Guizzardi S, Causa F, Giunti A. Osteoblast growth and function in porous poly epsilon-caprolactone matrices for bone repair: a preliminary study. Biomaterials 2003;24:3815–3824.
86.Im SY, Cho SH, Hwang JH, Lee SJ. Growth factor releasing porous poly (epsilon-caprolactone)-chitosan matrices for enhanced bone regenerative therapy. Arch Pharm Res 2003;26:76–82.
87.Wang YW, Wu Q, Chen J, Chen GQ. Evaluation of threedimensional scaffolds made of blends of hydroxyapatite and
poly(3-hydroxybutyrate-co-3-hydroxyhexanoate) for bone reconstruction. Biomaterials 2005;26:899–904.
88.Yang M, Zhu S, Chen Y, Chang Z, Chen G, Gong Y, Zhao N, Zhang X. Studies on bone marrow stromal cells affinity of poly (3-hydroxybutyrate-co-3-hydroxyhexanoate). Biomaterials 2004;25:1365–1373.
89.Kose GT, Kenar H, Hasirci N, Hasirci V. Macroporous poly(3- hydroxybutyrate-co-3-hydroxyvalerate) matrices for bone tissue engineering. Biomaterials 2003;24:1949–1958.
90.Farso NF, Karring T, Gogolewski S. Biodegradable guide for bone regeneration. Polyurethane membranes tested in rabbit radius defects. Acta Orthop Scand 1992;63:66–69.
91.Gorna K, Gogolewski S. Preparation, degradation, and calcification of biodegradable polyurethane foams for bone graft substitutes. J Biomed Mater Res 2003;67A:813–827.
92.Grad S, Kupcsik L, Gorna K, Gogolewski S, Alini M. The use of biodegradable polyurethane scaffolds for cartilage tissue engineering: potential and limitations. Biomaterials 2003; 24:5163–5171.
93.Warrer K, Karring T, Nyman S, Gogolewski S. Guided tissue regeneration using biodegradable membranes of polylactic acid or polyurethane. J Clin Periodontol 1992;19:633–640.
94.Ambrosio AM, Sahota JS, Runge C, Kurtz SM, Lakshmi S, Allcock HR, Laurencin CT. Novel polyphosphazenehydroxyapatite composites as biomaterials. IEEE Eng Med Biol Mag 2003;22:18–26.
95.Laurencin CT, El Amin SF, Ibim SE, Willoughby DA, Attawia M, Allcock HR, Ambrosio AA. A highly porous 3-dimensional polyphosphazene polymer matrix for skeletal tissue regeneration. J Biomed Mater Res 1996;30:133–138.
96.Laurencin CT, Norman ME, Elgendy HM, El Amin SF, Allcock HR, Pucher SR, Ambrosio AA. Use of polyphosphazenes for skeletal tissue regeneration. J Biomed Mater Res 1993;27:963–973.
97.Coetzee AS. Regeneration of bone in the presence of calcium sulfate. Arch Otolaryngol 1980;106:405–409.
98.Gitelis S, Piasecki P, Turner T, Haggard W, Charters J, Urban R. Use of a calcium sulfate-based bone graft substitute for benign bone lesions. Orthopedics 2001;24:162–166.
99.Ladd AL, Pliam NB. Use of bone-graft substitutes in distal radius fractures. J Am Acad Orthop Surg 1999;7:279–290.
100.Guarnieri R, Pecora G, Fini M, Aldini NN, Giardino R, Orsini G, Piattelli A. Medical grade calcium sulfate hemihydrate in healing of human extraction sockets: Clinical and histological observations at 3 months. J Periodontol 2004;75:902–908.
101.Kelly CM, Wilkins RM. Treatment of benign bone lesions with an injectable calcium sulfate-based bone graft substitute. Orthopedics 2004;27:s131–s135.
102.Urban RM, Turner TM, Hall DJ, Infanger S, Cheema N, Lim TH. Healing of large defects treated with calcium sulfate pellets containing demineralized bone matrix particles. Orthopedics 2003;26:s581–s585.
103.Turner TM, Urban RM, Hall DJ, Infanger S, Gitelis S, Petersen DW, Haggard WO. Osseous healing using injectable calcium sulfate-based putty for the delivery of demineralized bone matrix and cancellous bone chips. Orthopedics 2003;26:s571–s575.
104.Thalgott JS, Giuffre JM, Fritts K, Timlin M, Klezl Z. Instrumented posterolateral lumbar fusion using coralline hydroxyapatite with or without demineralized bone matrix, as an adjunct to autologous bone. Spine J 2001;1:131–137.
105.McConnell JR, Freeman BJ, Debnath UK, Grevitt MP, Prince HG, Webb JK. A prospective randomized comparison of coralline hydroxyapatite with autograft in cervical interbody fusion. Spine 2003;28:317–323.
106.Thalgott JS, Klezl Z, Timlin M, Giuffre JM. Anterior lumbar interbody fusion with processed sea coral (coralline