
- •VOLUME 1
- •CONTRIBUTOR LIST
- •PREFACE
- •LIST OF ARTICLES
- •ABBREVIATIONS AND ACRONYMS
- •CONVERSION FACTORS AND UNIT SYMBOLS
- •ABLATION.
- •ABSORBABLE BIOMATERIALS.
- •ACRYLIC BONE CEMENT.
- •ACTINOTHERAPY.
- •ADOPTIVE IMMUNOTHERAPY.
- •AFFINITY CHROMATOGRAPHY.
- •ALLOYS, SHAPE MEMORY
- •AMBULATORY MONITORING
- •ANALYTICAL METHODS, AUTOMATED
- •ANALYZER, OXYGEN.
- •ANESTHESIA MACHINES
- •ANESTHESIA MONITORING.
- •ANESTHESIA, COMPUTERS IN
- •ANGER CAMERA
- •ANGIOPLASTY.
- •ANORECTAL MANOMETRY
- •ANTIBODIES, MONOCLONAL.
- •APNEA DETECTION.
- •ARRHYTHMIA, TREATMENT.
- •ARRHYTHMIA ANALYSIS, AUTOMATED
- •ARTERIAL TONOMETRY.
- •ARTIFICIAL BLOOD.
- •ARTIFICIAL HEART.
- •ARTIFICIAL HEART VALVE.
- •ARTIFICIAL HIP JOINTS.
- •ARTIFICIAL LARYNX.
- •ARTIFICIAL PANCREAS.
- •ARTERIES, ELASTIC PROPERTIES OF
- •ASSISTIVE DEVICES FOR THE DISABLED.
- •ATOMIC ABSORPTION SPECTROMETRY.
- •AUDIOMETRY
- •BACTERIAL DETECTION SYSTEMS.
- •BALLOON PUMP.
- •BANKED BLOOD.
- •BAROTRAUMA.
- •BARRIER CONTRACEPTIVE DEVICES.
- •BIOCERAMICS.
- •BIOCOMPATIBILITY OF MATERIALS
- •BIOELECTRODES
- •BIOFEEDBACK
- •BIOHEAT TRANSFER
- •BIOIMPEDANCE IN CARDIOVASCULAR MEDICINE
- •BIOINFORMATICS
- •BIOLOGIC THERAPY.
- •BIOMAGNETISM
- •BIOMATERIALS, ABSORBABLE
- •BIOMATERIALS: AN OVERVIEW
- •BIOMATERIALS: BIOCERAMICS
- •BIOMATERIALS: CARBON
- •BIOMATERIALS CORROSION AND WEAR OF
- •BIOMATERIALS FOR DENTISTRY
- •BIOMATERIALS, POLYMERS
- •BIOMATERIALS, SURFACE PROPERTIES OF
- •BIOMATERIALS, TESTING AND STRUCTURAL PROPERTIES OF
- •BIOMATERIALS: TISSUE-ENGINEERING AND SCAFFOLDS
- •BIOMECHANICS OF EXERCISE FITNESS
- •BIOMECHANICS OF JOINTS.
- •BIOMECHANICS OF SCOLIOSIS.
- •BIOMECHANICS OF SKIN.
- •BIOMECHANICS OF THE HUMAN SPINE.
- •BIOMECHANICS OF TOOTH AND JAW.
- •BIOMEDICAL ENGINEERING EDUCATION
- •BIOSURFACE ENGINEERING
- •BIOSENSORS.
- •BIOTELEMETRY
- •BIRTH CONTROL.
- •BLEEDING, GASTROINTESTINAL.
- •BLADDER DYSFUNCTION, NEUROSTIMULATION OF
- •BLIND AND VISUALLY IMPAIRED, ASSISTIVE TECHNOLOGY FOR
- •BLOOD BANKING.
- •BLOOD CELL COUNTERS.
- •BLOOD COLLECTION AND PROCESSING
- •BLOOD FLOW.
- •BLOOD GAS MEASUREMENTS
- •BLOOD PRESSURE MEASUREMENT
- •BLOOD PRESSURE, AUTOMATIC CONTROL OF
- •BLOOD RHEOLOGY
- •BLOOD, ARTIFICIAL
- •BONDING, ENAMEL.
- •BONE AND TEETH, PROPERTIES OF
- •BONE CEMENT, ACRYLIC
- •BONE DENSITY MEASUREMENT
- •BORON NEUTRON CAPTURE THERAPY
- •BRACHYTHERAPY, HIGH DOSAGE RATE
- •BRACHYTHERAPY, INTRAVASCULAR
- •BRAIN ELECTRICAL ACTIVITY.
- •BURN WOUND COVERINGS.
- •BYPASS, CORONARY.
- •BYPASS, CARDIOPULMONARY.
11.Waples LM, Ropella KM. University partnerships in biomedical engineering. IEEE Eng Med Biol Mag 2003;22(4): 118–121.
12.The biomedical engineering curriculum database 2004. The Whitaker Foundation. Available at http://www.whitaker. org/academic/database/index.html. Accessed 2004 Nov. 18.
13.VaNTH ERC curriculum project (2004, June 10). VaNTH ERC [Online]. Available at http://www.vanth.org/curriculum/. Accessed [2004 Nov. 18].
14.Bransford JD, Brown AL, Cocking RR, editors. How People Learn: Brain, Mind, Experience, and School. Washington: National Academy Press; 1999.
15.Harris TR, Bransford JD, Brophy SP. Roles for learning sciences and learning technologies in biomedical engineering education: A review of recent advances. Annu Rev Biomed Eng 2002;4:29–48.
16.Benkeser PJ, Newstetter WC. Integrating soft skills in a BME curriculum, Proc. 2004 ASEE Annu Conf. 2004, June. American Society for Engineering Education. Available at http://www.asee.org/about/events/conferences/search.cfm. Accessed 2004 Nov. 19.
See also BIOINFORMATICS; MEDICAL EDUCATION, COMPUTERS IN; MEDICAL ENGINEERING SOCIETIES AND ORGANIZATIONS.
BIOSURFACE ENGINEERING
PETER MOLNAR
MELISSA HIRSCH-KUCHMA
JOHN W. RUMSEY
KERRY WILSON
JAMES J. HICKMAN
University of Central Florida
Orlando, Florida
INTRODUCTION
One primary reason there is a tremendous amount of interest in cellular patterning techniques is that numerous examples in nature use these techniques to segregate cells into tissues, vessels, and organs. The idea of templates in nature abounds for the creation of organized biological systems using both inorganic (1), as well as organic template systems (2). There is also a certain allure to being able to integrate electrically active cells directly to electronic devices using standard electronic fabrication techniques. Researchers have attempted to use surface cues to pattern cells since as early as 1917, in which spider webs were used to pattern cells (3). Most of the early work on cellular patterning used topographical cues until 1988, when a landmark publication by Kleinfeld et al. (4) used lithographic templating to fabricate simple patterns of cortical neurons. This was an adaptation of standard technology developed by the electronics industry to create computer chips that was then applied to the creation of patterns to guide neuronal cell attachment. It was at this point that interest in this field mushroomed, as the idea of creating neuronal networks from living neurons has potential applications in understanding biological information processing, creating hybrid computer systems, as well as a whole host of biomedical applications. Some prominent
BIOSURFACE ENGINEERING |
409 |
efforts to use cell patterning have been for spinal cord repair, creation of in vitro test bed systems to study diseases, as well as blood vessel formation from patterned endothelial cells (5). The initial lithography-based technique used by Kleinfeld et al. has been extended to include many other methods for the patterning of cells, including self-assembled monolayer (SAM) patterning, laser ablation, microcontact printing or stamping, ink jet printing, AFM printing, as well as patterning using microfluidic networks. Methods have also been extended in the area of topographical cues for 3D patterning, which has evolved from early work that used scratched grooves in glass surfaces. At this point, depending on the facilities that one has available, some form of mask making and pattern templating is available to just about any laboratory in the world. However, the biological interactions with these patterns that have been created are still not well developed, and this limits applications at this point.
There are many reasons for the lack of long-term applications of this technique, even after the large amount of work that has been done in this area. The first is that, in many instances, the patterns direct the initial attachment of cells, but as the extracellular matrix is deposited by the cells, long-term adherence to the patterns is not maintained. Another issue is that longer term cell survival is also dependent on factors besides the surface, such as media composition, cell–cell contact interactions, and the lack of growth factors that are normally present from other support cells and tissues. Defined systems are being developed in an attempt to control these other variables in addition to the surface (6,7), but these efforts have been limited to date. However, as progress is made in these areas, it will open up possible applications in tissue engineering, tissue repair, biosensors, and functional in vitro test beds.
PATTERNING METHODS
Many methods have been developed for creating templates to be used for cell patterning. These can be divided roughly into two categories: those that are derived from photolithography techniques and those that depend on physical segregation, although there is some crossover in the methods between the two. The photolithography-based systems typically use some sort of organic layer, from polymers to monolayers, which is illuminated, either directly with a pattern or through the template pattern to be created. This can involve many or a few steps depending on the particular method used. Generally, a second layer is deposited in the area where material was removed. Specific variations of this technique are discussed in this section.
The second major category, physical segregation, involves the actual placement of the molecules or cells in a pattern on a surface. Stamping is the most well-known method of creating a molecular-based template and of all the techniques is probably the most economical, but other techniques have been investigated for physical placement of cells in a desired pattern on the surface. Finally, both of these methods, which are 2D in nature, are now being extended into 3D patterning using many of the same
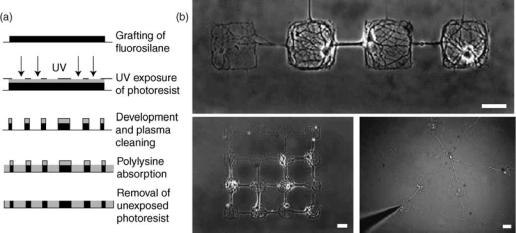
410 BIOSURFACE ENGINEERING
Figure 1. Protocol and images of the pattern. (a) Protocol of photolithography. A glass coverslip (continuous line) is coated with domains of fluorosilane molecule (dark) and with regions of polylysine (light gray) according to the pattern designed on the mask (dashed line), using ultraviolet (UV) exposure of a spin-coated photoresist (gray). (b) Images of neural networks of controlled architecture. Top: linear network. Bottom left: matrix 4 4. Bottom right: star. Cell bodies of neurons are restricted to squares or disks of 80 mm and neurites to line (80 mm length, 2–4 mm wide). Square and disk diameters are 80 mm for each figure. Scale bar is 50 mm (Ref. 9).
techniques, or combinations thereof, described therein. Below there is a brief description, along with the appropriate references, of the techniques that can be used to create these cellular templates.
Photolithography
A variety of photolithographic techniques has been employed to pattern proteins and cells on surfaces from the micrometer to the nanometer scale (8). The basic tools needed are a radiation source and a photomask. The photomask can be created using standard photolithography processes developed for the electronics industry. Irradiation of the surface through the photomask is used to create the patterns by ablation or by using a photosensitive material such as a photoresist as shown in Fig. 1.
Kleinfeld et al. (4) first demonstrated that dissociated neurons could be grown on 2D substrates consisting of lithographically defined patterned monolayers of diamines and triamines with alkylsilanes. The method used by Kleinfeld et al. started with a clean silicon or quartz surface that was spin coated with a layer of photoresist (an organic photosensitive polymer used in the electronics industry). The resist was exposed to UV light with a patterned photomask and then developed. The surface was refluxed in the presence of an alkylsilane, and the photoresist was then stripped off so that areas the photomask covered were reduced to bare silicon or quartz. These areas were then reacted with an aminosilane to form the patterned surface. The patterned cells developed electrical excitability and immunoreactivity for neuron-specific proteins. A further modification of this technique eliminated the photoresist from the pattern formation by direct ablation of the SAM layer (10).
Patterns of self-assembled monolayers formed from organosilanes on glass or silicon substrates and on gold
surfaces can be made by using a photoresist mask and deep UV radiation (10–18). Monolayers can also be directly ablated with various forms of radiation such as UV, X ray, ions, and electrons (8) depending on the resolution needed for the patterns. Organosilanes self-assemble and condense onto substrates that have surface –OH functionalities (19). The –SH functionality of the alkanethiol (20) is also highly reactive to ozone and other irradiation sources and has been used in patterning (21). Methods using X-ray or extreme UV (EUV) radiation give better resolution than the traditional photolithography using deep UV and photoresist masks. The ablated regions of the SAM can then be reacted with an organosilane or alkanethiol with different characteristics from the original layer to enable cell growth (22). Azides (23) and aromatic hydrocarbon silanes (24) have also been shown to be reactive for creating patterns.
A typical method to prepare a patterned glass silanated surface is illustrated next. The glass must first be acid cleaned or oxygen plasma cleaned to maximize the surface
–OH functional density. Next, the glass is reacted with a silane that contains -chloro, -methoxy, or -ethoxy bonds in the presence of a small amount of water that acts as a catalyst. A mask is then used to protect certain areas of the surface while allowing the radiation source to ablate others in the desired pattern. The ablated regions of the surface can then be coated with a different silane with different properties than the original, thus forming the patterned surface; an example of this is shown in Fig. 2.
The photoreactivity of polymers, such as poly(ethylene) glycol and polystyrene, has also been used to pattern surfaces (25). Biologically based polymers, such as poly- L-lysine and extracellular matrix proteins, have been ablated tso create patterns (26). Polymer photolithography followed by protein adsorption has been combined to create patterned cytophobic and cytophilic areas. Patterning of perfluoropolymers followed by adsorption of poly-L-lysine
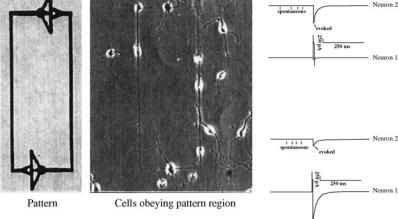
BIOSURFACE ENGINEERING |
411 |
and albumin has been one method used (27). Photosensitive polymers have been treated with UV radiation to create an –COOH functional unit on the surface and then patterned via the linkage of proteins to form cytophilic and cytophobic regions (28). A bioactive photoresist (bioresist) has been developed that does not require the use of solvents that can denature the biomolecule that is patterned (29).
Photolithographic protein patterning requires the attachment of photosensitive groups to proteins on a substrate. Patterns can be made using a patterned mask and selective ablation. This method has been shown to be useful to produce micropatterned cultures (30). Various methods are used to covalently attach proteins in patterns to surfaces (31), to pattern using biomolecule photoimmobilization (32), and to create density gradients of photoreactive biomolecules (33). Heterobifunctional crosslinker molecules have been used to attach proteins to silanated surfaces both before and after the photolithographic patterning step (34). Protein patterning has been achieved using a micromirror array (MMA), which can transfer a pattern from the mirrors that are switched on, ablating a photolabile protecting group (35). Photolithography was also used to pattern thermosensitive copolymers through polymer grafting (36). The surface micropattern appeared and disappeared interchangeably, as observed under a phase-contrast microscope, by varying the temperature between 10 and 37 8C. The copolymer-grafted polystyrene surface was hydrophobic at 37 8C and hydrophilic at 10 8C.
Photolithography provides high resolution patterning and the ability to make complex patterns on surfaces. Unlike stamping techniques, the patterns are more permanent; however, the process can be relatively expensive as it generally requires the use of a laser and clean room facilities for the mask production. To attach proteins, such as ECM proteins, the use of a covalently attached crosslinker is necessary, and stamping techniques are generally preferred for this application.
Microcontact Printing (Stamping)
Microcontact printing was introduced by George Whiteside’s group at Harvard in 1994 (37) to pattern selfassembled monolayers on gold substrates to control surface properties, cell adhesion, proliferation, and protein secre-
Figure 2. Micrograph of circuit-patterned day 2 in vitro hippocampal neurons plated onto DETA/ 15F modified glass coverslips. Electrophysiology of day 12 in vitro hippocampal neurons displaying both spontaneous and evoked activity on a DETA/15F line-space patterned surface. Top trace: post-synaptic neuron. Bottom trace: stimulated presynaptic neuron.
tion by patterned cells. The basic method to create surface patterns by microcontact printing has not changed much since then. Usually, a poly(dimethylsiloxane) (PDMS) stamp is created using a molding technique from a master pattern relief mold and then used to transfer chemical patterns to flat or curved surfaces. The master is usually prepared from silicon by standard photolithography and/or etching, but other substrates can also be used. The transferred chemical patterns can be created using a compound that binds covalently to the substrate (e.g., selfassembled monolayers or proteins immobilized by crosslinkers) (38) or a compound that binds noncovalently, such as absorbed extracellular matrix proteins (39). This methodology is illustrated in Fig. 3. Oliva et al. presented a novel method to couple proteins to patterned surfaces based on the strong interaction of protein A and the Fc fragment of immunoglobulins. This method involved the creation of a covalently coupled Fc fragment and the target protein (41). Methods have also been developed to transfer proteins from a fluid phase to a surface using hydrogels as the stamp (42). Moreover, recently introduced techniques are allowing the creation of protein gradients with microcontact printing (43). Although alignment of the stamp/patterns with surface features such as microelectrodes is more difficult than in the case of photolithographic patterning, several groups are beginning to address this issue (44). Microcontact printing is usually a favored method among biologists compared with photolithography, because (1) the equipment and controlled environment facilities required for photolithography are not routinely available to cell biologists and (2) the steps are simpler to pattern proteins, the molecules of greatest interest to biologists, using microcontact printing than with photolithography and crosslinkers. The refinement of the PDMS molding technique has directly led to the development of another important patterning method, microfluidics, which gained wider applications with the introduction of microelectromechanical systems (MEMS) and ‘‘lab-on-a- chip’’ systems. However, initial results using PDMS indicated some transfer of the PDMS to the surface during the stamping process. This can be troublesome in cell patterning applications as PDMS can be toxic to cells or mask the chemical functionality of interest. Methods of ‘‘curing’’ the stamps or presoaking to enable better release of the compound has been reported (45).
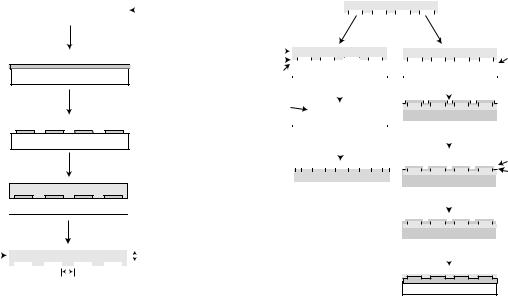
412 BIOSURFACE ENGINEERING
Si |
|
|
Silicon wafer |
|
|
Spin coat photoresist
Photoresist
Si
Expose to UV light through a mask and then expose to a solution of developer
"Master"
Si
Cast PDMS
PDMS
Si
Remove PDMS from master
"Stamp" |
|
|
|
|
|
|
|
|
PDMS |
|
|
|
100 µm - 1 cm |
||||||||||||
|
|
|
|
|
|
|
|||||||||||||||||||
|
|
|
|
|
|
|
|
|
|
|
|
|
|
|
|
|
|
|
|
|
|
|
|
|
1 µm - 500 µm |
|
|
|
|
|
|
|
|
|
|
|
|
|
|
|
|
|
|
|
|
|
|
|
|
|
Features:
~500 nm - 500 µm
|
|
"Stamp" |
|
|
|
|
|
|
|
PDMS |
|
|
|
|
|
|
|
|
|
|
|
|||||||||
|
1. Ink stamp with |
|
|
|
|
|
|
|
|
|
|
|
Mold polyurethane |
|||||||||||||||||
|
|
|
|
|
|
|
|
|
|
|
||||||||||||||||||||
|
alkanethiol |
|
|
|
|
|
|
A. |
B. |
|
|
|
||||||||||||||||||
|
|
|
|
|
|
|
|
|
|
against PDMS |
|
|||||||||||||||||||
|
2. Place stamp |
|
|
|
|
|
|
|
|
|
|
|
|
|
|
|
||||||||||||||
Stamp |
on metal |
|
|
|
|
|
|
|
|
|
|
|
|
|
|
|
|
|
|
|
|
|
|
|
|
|||||
|
|
|
|
|
|
|
PDMS |
|
|
|
|
|
|
|
PDMS |
|
Polyurethane |
|||||||||||||
Alkanethiol |
|
|
|
|
|
|
|
|
|
|
|
|
|
|
|
|
|
|
|
|
|
|
|
|
|
|
|
|||
Gold or silver |
|
|
Si or Glass |
|
|
|
|
|
|
|
|
Glass |
|
|
||||||||||||||||
|
|
|
|
|
|
|
|
|
|
|
Remove stamp |
|
|
|
|
|
1. Remove PDMS |
|||||||||||||
|
|
|
|
|
|
|
|
|
|
|
|
|
|
|
|
|||||||||||||||
|
|
|
|
|
|
|
|
|
|
|
|
|
|
|
|
2. Evaporate Au or Ag |
||||||||||||||
Patterned SAM |
|
|
|
|
|
|
|
|
|
|
|
|
|
|
|
|
|
|
|
|
|
|
|
|
||||||
|
|
|
|
|
|
|
|
|
|
|
|
|
|
|
|
|
|
|
|
|
|
|
|
|||||||
|
|
|
|
|
|
|
|
|
|
|
|
|
|
|
|
|
|
|
|
|
|
Glass |
|
|
||||||
|
|
|
|
|
|
|
Si or Glass |
|
|
|
|
|
|
|
|
|||||||||||||||
|
|
|
|
|
|
|
|
|
|
|
|
|
|
Place stamp |
|
|||||||||||||||
|
|
|
|
|
|
|
|
|
|
|
|
|
|
|
|
|
|
|
|
|
|
|
|
|
|
|||||
|
|
|
|
|
|
|
|
|
|
|
Wash with other |
|
|
|
|
|
on metal |
|
||||||||||||
|
|
|
|
|
|
|
|
|
|
|
|
|
|
|
|
|
||||||||||||||
|
|
|
|
|
|
|
|
|
|
|
alkanethiol |
|
|
|
|
|
|
|
|
|
|
|
|
|||||||
|
|
|
|
|
|
|
|
|
|
|
|
|
PDMS |
|
|
|
|
Alkanethiol |
||||||||||||
|
|
|
|
|
|
|
|
|
|
|
|
|
|
|
|
|
|
|
|
|
|
|
|
|
|
|||||
|
|
|
|
|
|
|
|
|
|
|
|
|
|
|
|
|
|
|
|
|
|
|
|
|
|
|
|
|
|
Gold or silver |
|
|
|
|
|
|
|
Si or Glass |
|
|
|
|
|
|
Glass |
|
|
||||||||||||||
|
|
|
|
|
|
|
|
|
|
|
|
|
|
|
|
|
|
|
|
|||||||||||
|
|
|
|
|
|
|
|
|
|
|
|
|
|
|
|
|
|
|
|
|
|
|
|
|
Remove stamp |
|||||
|
|
|
|
|
|
|
|
|
|
|
|
|
|
|
|
|
|
|
|
|
|
|
|
|
||||||
|
|
|
|
|
|
|
|
|
|
|
|
|
|
|
|
|
|
|
|
|
|
|
|
|
|
|
|
|
||
|
|
|
|
|
|
|
|
|
|
|
|
|
|
|
|
|
|
|
|
|
|
|
|
|
|
|
|
|
|
|
|
|
|
|
|
|
|
|
|
|
|
|
|
|
|
|
|
|
|
|
|
|
|
|
|
|
|
|
|
|
|
|
|
|
|
|
|
|
|
|
|
|
|
|
|
|
|
|
|
|
|
|
|
|
|
|
|
|
|
|
|
|
|
|
|
|
|
|
|
|
|
|
|
|
|
|
|
|
|
|
|
|
|
|
Glass |
|
|
||||||
|
|
|
|
|
|
|
|
|
|
|
|
|
|
|
|
|
|
|
|
|
|
|
|
|
Wash with other |
|||||
|
|
|
|
|
|
|
|
|
|
|
|
|
|
|
|
|
|
|
|
|
|
|
|
|
||||||
|
|
|
|
|
|
|
|
|
|
|
|
|
|
|
|
|
|
|
|
|
|
|
|
|
alkanethiol |
|
||||
|
|
|
|
|
|
|
|
|
|
|
|
|
|
|
|
|
|
|
|
|
|
|
|
|
|
|
|
|
|
|
Glass
Figure 3. The creation of the master stamp is indicated on the left side of the figure, and its use to make patterns is indicated on the flow chart to the right (40).
Inkjet Printing
Inkjet technology used in desktop printers can be applied to the creation of viable cell patterns by printing proteins on to a surface (46). It is a fast and inexpensive method that does not require any contact (such as with stamping) to the surface. This method is desirable for high throughput printing of surfaces, and there is good control of the drop volume and of the alignment of the pattern. Printing occurs when small volumes of a protein solution or a solution containing cells is pumped through a nozzle in a continuous jet or small droplets of the solution are formed either by an acoustic or thermal pulse. The drop size is in the range of 10–20 pL (8). Inkjet printing and the use of computer-aided design (CAD) have impacted the biomaterials field greatly in the areas of biosensor development (47), immobilization of bacteria on biochips (48), DNA arrays and synthesis (49), microdeposition of proteins on cellulose (50), and free-form fabrication techniques to create acellular polymeric scaffolds. A drawback of this method is that the resolution, in the 20–50 mm range, is limited by the statistical variations in the drop direction and spreading on the surface (8). Other high throughput printing methods have also been adapted to pattern proteins on surfaces for biological application such as the already developed DNA spotter used to create DNA microchips (51).
Patterning via Microfluidic Networks
Synthetic surfaces may also be patterned using microfluidic networks (mFNs) to selectively generate regions with greater cytophilicity. This method involves the use of a microfluidic network fabricated in an elastomeric polymer, usually polydimethylsiloxane (PDMS), to direct a protein solution to the regions where cell-adhesion is desired. Gravity and pressure-driven flows are the most common methods for circulating the solution.
The most basic application of this method involves allowing a solution of the material to be patterned noncovalently to the substrate surface using the microchannels as guides. Some of the earliest work done with this method by Folch and Toner (52) involved patterning of various polymer surfaces with human plasma fibronectin (Fn) and collagen to create adhesion promoting domains for hepatocyte/fibroblast cocultures. Similar work by Chiu et al. (53) involved the patterning of glass coverslips with fibrinogen (Fb) and bovine serum albumin (BSA) for patterned cocultures with bovine adrenal capillary endothelial cells (BCEs) and human bladder cancer cells (ECVs). Further work by Takayama et al. (54) demonstrated an added degree of sophistication of this technique by using the laminar flow characteristics of microchannels to generate patterns using a single microchannel.
In addition to simple noncovalent binding of proteins to the substrate, it is possible to use a crosslinking agent to covalently link a molecule of interest. Delamarche et al. (55) used a hydroxylsuccinimidyl ester to chemically couple immunoglobulin G (IgG) to various substrates. Another more commonly used method involves functionalized silane SAMs on substrates, such as 3-aminopropyltriethox- ysilane (APTES), and a crosslinking reagent, such glutaraldehyde (GA), to achieve crosslinking of protein molecules to a substrate. Romanova et al. (25) demonstrated the applicability of this method using microfluidic patterning to study controlled growth of Aplysia neurons on geometric patterns of poly-L-lysing and collagen IV. Yet another variation on this method was developed by Itoga et al. (56), who generated patterns by photopolymerization of acrylamide on 3-methacryloxypropyltrimethoxysilane modified glass coverslips. In this method, the acrylamide monomer was flowed through microchannels adhered to the derivatized coverslip and cross-linked via photopolymerization to generate cytopholic poly-acrylamide regions.
Perhaps the most sophisticated application of microfluidic patterning of substrates for cell adhesion was demonstrated by Tan and Desai (57,58), who demonstrated the fabrication of complex multilayer cocultures for biomimetic blood vessels. In this work, the 3D structure of blood vessels was recreated by differential deposition of protein and cell layers on a glass coverslip. By alternately layering proteins (Collagen I, collagen/chitosan, and matrigel) and cell types found in blood vessels (fibroblasts, smooth muscle, and endothelial cells), it was possible to recreate layers mimicking the adventitial, medial, and intimal layers observed in blood vessels.
Topographical (3D) Patterning Methods
Control of Cell Placement, Movement, and Process Growth Based on Topographical Clues. It has been known for many years that cells react to topographical clues in their environment (59). Originally, natural fibers were used to create topological clues. Later, fabrication methods developed for the microchip industry were adapted to micromachine silicon surfaces for cell culture applications (45). For the creation of microor nanotopography, sophisticated methods and equipment are necessary, which are available in most electronics laboratories, but are usually not available to cell biologists. In recent years, as a response to the increased need for highthroughput screening methods (planar patch clamp, lab-on-a-chip) and in response to the challenge of biological applications of nanoscience, several multidisciplinary team/centers have been established with microfabrication capabilities. The most commonly used fabrication methods are (1) silicon etching (60), (2) photo- resist-based methods (61), (3) PDMS molding (62), and (4) polymer/hydrogel molding (63). Methods have also been developed for the creation of complex 3D structures by the rapid prototyping/layer-by-layer technique (58). Tan et al. (62) used 3D PDMS structures not only to control attachment and morphology of cells but also to measure attachment force through flexible microneedles as the culture substrate. Xi et al. used AFM cantilevers to demonstrate and measure the contracting force of cardiac muscle cells (64).
3D Patterning of Living Cells. Several hydrogel scaffoldbased methods have also been developed to create 3D patterns from cells. For example, photo-polymerization of hydrogels can be used to create patterns of entrapped cells (65). These scaffolding methods offer possible intervention in spinal cord injury (66). Layer-by-layer methods have also been used to create complex ‘‘tissue analog’’ cellular structures, such as blood vessels (57).
Other Patterning Methods
Several other methods, based on microcontact printing and PDMS stamping, have been developed to create cellular patterns. Folch et al. used an inexpensive method to create microwells for coculture experiments based on a reusable elastomeric stencil (i.e., a membrane containing thru holes), which seals spontaneously against the surface (67,68). Gole and Sastry developed a novel method to pattern surfaces with lipids followed by selective protein
BIOSURFACE ENGINEERING |
413 |
incorporation into the lipid patterns that result in complex protein patterns on the surface (69). A scanning electrochemical microscope has also been adapted to pattern selfassembled monolayers on surfaces with high spatial resolution by either chemical removal of SAMs (70) or by gold deposition (71). Amro et al. used an AFM tip to directly print nanoscale patterns using the so-called dip-pen technique (72–74). However, none of these methods has been proven beyond the demonstration step.
Applications
Many potential applications for cell patterning remain in the biomedical and biotechnology fields. However, there has been limited success to date, besides demonstrations in the literature, for any of the applications envisioned by the host of researchers in this area. However, the promise of the use of cellular patterning for real applications is bolstered by the success that has been achieved for patterning of DNA, RNA, and protein arrays (42,51) as well as for enzymatic biosensors, such as simple pregnancy tests. Much like with cellular patterning, there was a period of time during the development of molecular patterning techniques before the applications became relevant, and the authors believe this is the situation that exists for cell patterning at this time. One reason for this long development stage is that viable and reproducible cellular patterns have many other variables that are not a major issue with biomolecule patterning applications. The cellular media are very important for cell survival, especially long term, as well as cell preparation, which has a significant affect on an extracellular attachment. In addition, no universal combination will be good for every cell, as each cell type has a unique environment that it needs to survive and function, and some aspect of these factors needs to be reproduced for long-term applications. That said, many examples of tissues exhibit some segregation, including blood vessels, lung tissue, the lining of the stomach and intestines, as well as a host of other tissues, which could benefit from this methodology. However, one of the most studied cell tissues is that of the central nervous system (CNS), which exhibits a complicated network of structures that will be difficult to reproduce for reconstruction or repair of neuronal tissue or for other in vivo as well as in vitro applications. To date, there has been some success in manipulating cells in patterns and controlling certain variables that would be necessary for the creation of functional tissues, but a complete system, using this methodology, has not been reported in the literature. However, there has been some success in demonstrating the intermediate steps that will be necessary for the realization of applications of this method for biomedical and biotechnological applications. Cell attachment has been demonstrated by several researchers, and generally, pattern adherence is maintained for approximately 1 week although longer times have been demonstrated (75). Control of cell morphology and differentiation are two important factors that are necessary in creating functional systems, and these have also been demonstrated. For neuronal-based systems, the primary variable, that of axonal polarity, has also been demonstrated. Brief descriptions and progress in these areas are described below.
414 BIOSURFACE ENGINEERING
Controlling Cell Attachment, Morphology, and Differentiation. In vivo, cells are arranged in distinct patterns (76). This patterning effect is dictated during development, with cues provided by both physical contact with other cells and chemicals present in the extracellular matrix (77). Because the random arrangement of cells cultured in vitro does not represent the complex architecture seen in tissues, studies of many cell types lack a clear in vivo relationship. Consequently, techniques to create defined and reproducible functional patterns of cells on surfaces have been created.
Controlling Attachment and Morphology. Several methods have been developed to control the attachment of cells on surfaces creating patterns that more accurately mimic conditions found in vivo. Using photolithography, microcontact printing, and microstamping, groups have been able create 2D patterns that guide cell attachment and alignment (37,78–81). Three-dimensional patterning techniques have also been employed to influence cell orientation and polarity of neurons and osteoblasts (82–84). Cells have also been attached to capillaries and microfluidic devices using SAMs and protein adsorption or microcontact printing (85,86). Additionally, cell attachment and proliferation has been enhanced using biomolecules attached covalently, by stamping, or microcontact printing to surfaces (37,78–82,84–87).
Controlling Morphology and Differentiation of Cell Types Other Than Neurons. Microfabrication and photolithography used to create microtextured membranes for cardiac myocyte culture showed greater levels of attachment and cell height relative to 2D culture techniques (88). Similar techniques applied to vascular smooth muscle also showed the ability to control shape and size of the cells (89). Furthermore, microtextured surfaces were shown to influence gene expression and protein localization in neonatal cardiomyocytes (90). Cues provided to cells by the topography of their extracellular environment are thought to play a role in differentiation. The generation of microtopographical surfaces in titanium has been used to regulate the differentiation of osteoblasts in vitro (91).
Study of Axon Guidance in Neurons. Using photolithographic techniques and SAMs, the 2D patterns created were shown to influence neuronal polarity (22). Photolithographically fabricated 3D surfaces demonstrated that topology also influenced the orientation of neurons and the polarity of axonal outgrowth (83). The ability to guide neurite outgrowth and axonal elongation has significant applications in the areas of spinal cord repair, synapse, formation, and neural network formation. Initial studies using striped patterns on glass coverslips showed that neurons would adhere and preferentially extend axons along the length of the pattern (4,92). Growth of neurons on micropatterned 2D surfaces showed preferential axon extension along the length of the pattern as well as increased axon extension (92–96).
The use of 3D microchannels and microstructured surfaces has also been shown to increase the complexity of neuronal architecture, increase neurite growth, and enhance cell activity (61). Photolithography has also been
used to pattern neurons and control axon elongation for the formation of neuronal networks (25,97). The synapses formed by these hippocampal neurons showed strong electrophysiological activity up to 17 days in culture (10). These network formations show promise for use in screening pharmacological agents as well as for electronic connection.
BIBLIOGRAPHY
Cited References
1.Fritz M, Belcher AM, Radmacher M, Walters DA, Hansma PK, Stucky GD, Morse DE, Mann S. Flat pearls from biofabrication of organized composites on inorganic substrates. Nature Biotechnol 1994;371:49–51.
2.Noctor SC, Flint AC, Weissman TA, Dammerman RS, - Kriegstein AR. Neurons derived from radial glial cells establish radial units in neocortex. Nature 2001: 409(6821): 714–720.
3.Harrison RG. The reaction of embryonic cells to solid structures. J Exp Zool 1914;17:521–544.
4.Kleinfeld D, Kahler KH, Hockberger PE. Controlled outgrowth of dissociated neurons on patterned substrates. J Neurosci 1988;8(11):4098–4120.
5.Spargo BJ, Testoff MA, Nielsen TB, Stenger DA, Hickman JJ, Rudolph AS. Spatially controlled adhesion, spreading, and differentiation of endothelial-cells on self-assembled molecular monolayers. Proc Nat Acad Sci 1994;91(23):11070–11074.
6.Das M, Molnar P, Gregory C, Riedel L, Jamshidi A, Hickman
JJ.Long-term culture of embryonic rat cardiomyocytes on an organosilane surface in a serum-free medium. Biomaterials 2004;25(25):5643–5647.
7.Das M, Bhargava N, Gregory C, Riedel L, Molnar P, Hickman
JJ.Adult rat spinal cord culture on an organosilane surface in a novel serum-free medium. In Vitro Animal Cell Develop Bio 2005. In press.
8.Geissler M, Xia Y. Patterning: Principles and some new developments. Adv Mater 2004;16(15):1249–1269.
9.Wyart C, Ybert C, Bourdieu L, Herr C, Prinz C, Chatenay D. Constrained synaptic connectivity in functional mammalian neuronal networks grown on patterned surfaces. J Neurosci Methods 2002;117(2):123–131.
10.Dulcey CS, Georger JHJr. Krauthamer V, Stenger DA, Fare TL, Calvert JM. Deep UV photochemistry of chemisorbed monolayers: Patterned coplanar molecular assemblies. Science 1991;252(5005):551–554.
11.Dressick WJ, Calvert JM. Patterning of self-assembled films using lithographic exposure tools. Appl Phys Part 1 1993; 32(12B):5829–5839.
12.Bhatia SK, Teixeira JL, Anderson M, Shriver-Lake LC, Calvert JM, Georger JH, Hickman JJ, Dulcey CS, Schoen PE, Ligler FS. Fabrication of surfaces resistant to protein adsorption and application to two-dimensional protein patterning. Anal Biochem 1993;208(1):197–205.
13.Liu J, Hlady V. Chemical pattern on silica surface prepared by UV irradiation of 3-mercaptopropyltriethoxy silane layer: Surface characterization and fibrinogen adsorption. Colloids Surfaces B-Biointerfaces 1996;8(1–2):25–37.
14.Dressick WJ, Dulcey CS, Chen MS, Calvert JM. Photochemical studies of (aminoethylaminomethyl)phenethyltrimethoxysilane self-assembled monolayer films. Thin Solid Films 1996;285:568–572.
15.Georger JH, Stenger DA, Rudolph AS, Hickman JJ, Dulcey CS, Fare TL. Coplanar patterns of self-assembled monolayers for selective cell-adhesion and outgrowth. Thin Solid Films 1992;210(1–2):716–719.
16.Stenger DA, Georger JH, Dulcey CS, Hickman JJ, Rudolph AS, Nielsen TB, McCort SM, Calvert JM. Coplanar molecular assemblies of aminoalkylsilane and perfluorinated alkyl- silane—characterization and geometric definition of mam- malian-cell adhesion and growth. J Am Chem Soc 1992;114 (22):8435–8442.
17.Calvert JM. Lithographic patterning of self-assembled films. J Vacuum Sci Technol B 1993;11(6):2155–2163.
18.Ravenscroft MS, Bateman KE, Shaffer KM, Schessler HM, Jung DR, Schneider TW, Montgomery CB, Custer TL, Schaffner AE, Liu QY, Li YX, Barker JL, Hickman JJ. Developmental neurobiology implications from fabrication and analysis of hippocampal neuronal networks on patterned silanemodified surfaces. J Am Chem Soc 1998;120(47): 12169–12177.
19.Plueddemann EP. Silane adhesion promoters in coatings. Progr Organic Coatings 1983;11(3):297–308.
20.Whitesides GM, Laibinis P, Folkers J, Prime K, Seto C, Zerkowski J. Self-assembly—alkanethiolates on gold and hydrogen-bonded networks. Abstr Papers Am Chem Soc 1991;201:103-INOR.
21.Gillen G, Wight S, Bennett J, Tarlov MJ. Patterning of selfassembled alkanethiol monolayers on silver by microfocus ion and electron-beam bombardment. Appl Phys Lett 1994;65(5): 534–536.
22.Stenger DA, Hickman JJ, Bateman KE, Ravenscroft MS, Ma W, Pancrazio JJ, Shaffer K, Schaffner AE, Cribbs DH, Cotman CW. Microlithographic determination of axonal/dendritic polarity in cultured hippocampal neurons. J Neurosci Methods 1998;82(2):167–173.
23.Matsuda T, Sugawara T. Development of surface photochemical modification method for micropatterning of culturedcells. J Biomed Mater Res 1995;29(6):749–756.
24.Dulcey CS, Georger JH, Chen MS, McElvany SW, Oferrall CE, Benezra VI, Calvert JM. Photochemistry and patterning of self-assembled monolayer films containing aromatic hydrocarbon functional groups. Langmuir 1996;12(6):1638–1650.
25.Romanova EV, Fosser KA, Stanislav SR, Nuzzo RG, Sweedler JV. Engineering the morphology and electrophysiological parameters of cultured neurons by microfluidic surface patterning. FASEB J 2004.
26.Corey JM, Wheeler BC, Brewer GJ. Compliance of hippocampal neurons to patterned substrate networks. J Neurosci Res 1991;30(2):300–307.
27.Griscom L, Degenaar P, LePioufle B, Tamiya E, Fujita H. Techniques for patterning and guidance of primary culture neurons on micro-electrode arrays. Sen Actuators B 2002;83 (1–3):15–21.
28.Nicolau DV, Taguchi T, Taniguchi H, Tanigawa H, Yoshikawa S. Patterning neuronal and glia cells on lightassisted functionalised photoresists. Biosens Bioelectron 1999;14(3):317–325.
29.He W, Halberstadt CR, Gonsalves KE. Lithography application of a novel photoresist for patterning of cells. Biomaterials 2004;11:2055–8063.
30.Liu GY, Amro NA. Positioning protein molecules on surfaces: A nanoengineering approach to supramolecular chemistry. Proc Nat Acad Sci 2002;99(8):5165–5170.
31.Pirrung MC, Huang CY. A general method for the spatially defined immobilization of biomolecules on glass surfaces using ‘‘caged’’ biotin. Bioconjugate Chem 1996;7(3):317–321.
32.Sigrist H, Collioud A, Clemence JF, Gao H, Luginbuhl R, Sanger M, Sundarababu G. Surface immobilization of biomolecules by light. Opt Eng 1995;34(8):2339–2348.
33.Herbert CB, McLernon TL, Hypolite CL, Adams DN, Pikus L, Huang CC, Fields GB, Letourneau PC, Distefano MD, Hu WS. Micropatterning gradients and controlling surface densities of photoactivatable biomolecules on self-assembled
BIOSURFACE ENGINEERING |
415 |
monolayers of oligo(ethylene glycol) alkanethiolates. Chem Bio 1997;4(10): 731–737.
34.Sorribas H, Padeste C, Tiefenauer L. Photolithographic generation of protein micropatterns for neuron culture applications. Biomaterials 2002;23(3):893–900.
35.Lee K-N, Shin D-S, Lee Y-S, Kim Y-K. Protein patterning by virtual mask photolithography using a micromirror array. J Micromech Microeng 2003;13(1):18–25.
36.Chen GP, Imanishi Y, Ito Y. Effect of protein and cell behavior on pattern-grafted thermoresponsive polymer. J Biomed Mater Res 1998;42(1):38–44.
37.Singhvi R, Kumar A, Lopez GP, Stephanopoulos GN, Wang DI, Whitesides GM, Ingber DE. Engineering cell shape and function. Science 1994;264(5159):696–698.
38.Lahiri J, Ostuni E, Whitesides GM. Patterning ligands on reactive SAMs by microcontact printing. Langmuir 1999;15 (6):2055–2060.
39.Cornish T, Branch DW, Wheeler BC, Campanelli JT. Microcontact printing: A versatile technique for the study of synaptogenic molecules. Mol Cell Neurosci 2002;20(1):140–153.
40.Kane RS, Takayama S, Ostuni E, Ingber DE, Whitesides GM. Patterning proteins and cells using soft lithography. Biomaterials 1999;20(23–24):2363–2376.
41.Oliva AA, James CD, Kingman CE, Craighead HG, Banker GA. Patterning axonal guidance molecules using a novel strategy for microcontact printing. Neurochem Res 2003;28 (11):1639–1648.
42.Martin BD, Gaber BP, Patterson CH, Turner DC. Direct protein microarray fabrication using a hydrogel ‘‘stamper’’. Langmuir 1998;14(15):3971–3975.
43.Mayer M, Yang J, Gitlin I, Gracias DH, Whitesides GM. Micropatterned agarose gels for stamping arrays of proteins and gradients of proteins. Proteomics 2004;4(8):2366–2376.
44.Lauer L, Ingebrandt S, Scholl M, Offenhausser A. Aligned microcontact printing of biomolecules on microelectronic device surfaces. IEEE Trans Biomed Eng 2001;48(7):838– 842.
45.Craighead HG, James CD, Turner AMP. Chemical and topographical patterning for directed cell attachment. Curr Opin Solid State Mater Sci 2001;5(2–3):177–184.
46.Roth EA, Xu T, Das M, Gregory C, Hickman JJ, Boland T. Inkjet printing for high-throughput cell patterning. Biomaterials 2004;25(17):3707.
47.Newman JD, Turner APF, Marrazza G. Ink-jet printing for the fabrication of amperometric glucose biosensors. Anal Chim Acta 1992;262(1):13–17.
48.Xu T, Jin J, Gregory C, Hickman JJ, Boland T. Inkjet printing of viable mammalian cells. Biomaterials 2005;26(1):93–99.
49.Schena M, Heller RA, Thieriault TP, Konrad K, Lachenmeier E, Davis RW. Microarrays: Biotechnology’s discovery platform for functional genomics. Trends Biotechnol 1998;16(7):301–306.
50.Roda A, Guardigli M, Russo C, Pasini P, Baraldini M. Protein microdeposition using a conventional ink-jet printer. Biotechniques 2000;28(3):492–496.
51.Flaim CJ, Chien S, Bhatia SN. An extracellular matrix microarray for probing cellular differentiation. Nature Methods 2005;2(2):119–125.
52.Folch A, Toner M. Cellular micropatterns on biocompatible materials. Biotechnol Progr 1998;14(3):388–392.
53.Chiu DT, Jeon NL, Huang S, Kane RS, Wargo CJ, Choi IS, Ingber DE. Whitesides GM. Patterned deposition of cells and proteins onto surfaces by using three-dimensional microfluidic systems. Proc Natl Acad Sci USA 2000;97(6):2408– 2413.
54.Takayama S, McDonald JC, Ostuni E, Liang MN, Kenis PJA, Ismagilov RF, Whitesides GM. Patterning cells and their
416 BIOSURFACE ENGINEERING
environments using multiple laminar fluid flows in capillary networks. Proc Natl Acad Sci USA 1999;96(10):5545–5548.
55.Delamarche E, Bernard A, Schmid H, Michel B, Biebuyck H. Patterned delivery of immunoglobulins to surfaces using microfluidic networks. Science 1997;276(5313):779–781.
56.Itoga K, Yamamoto JK, Kikuchi A, Okano T. Micropatterned surfaces prepared using a liquid crystal projector-modified photopolymerization device and microfluidics. J Biomed Mater Res 2004;69A:391–397.
57.Tan W, Desai TA. Microscale multilayer cocultures for biomimetic blood vessels. J Biomed Mater Res 2005;72A(2):146– 160.
58.Tan W, Desai TA. Layer-by-layer microfluidics for biomimetic three-dimensional structures. Biomaterials 2004;25(7–8): 1355–1364.
59.Curtis A, Wilkinson C. Topographical control of cells. Biomaterials 1997;18(24):1573–1583.
60.Turner S, Kam L, Isaacson M, Craighead HG, Shain W, Turner J. Cell attachment on silicon nanostructures. J Vacuum Sci Technol B 1997;15(6):2848–2854.
61.Mahoney MJ, Chen RR, Tan J, Saltzman WM. The influence of microchannels on neurite growth and architecture. Biomaterials 2005;26(7):771–778.
62.Tan JL, Tien J, Pirone DM, Gray DS, Bhadriraju K, Chen CS. Cells lying on a bed of microneedles: An approach to isolate mechanical force. Proc Natl Acad Sci USA 2003;100(4):1484– 1489.
63.Recknor JB, Recknor JC, Sakaguchi DS, Mallapragadaa SK. Oriented astroglial cell growth on micropatterned polystyrene substrates. Biomaterials 2004;25(14):2753–2767.
64.Xi JZ, Schmidt J, Montemagno C. Development of selfassembled muscle-MEMS microdevices. Biophys J 2004;86 (1):481A.
65.Albrecht DR, Tsang VL, Sah RL, Bhatia SN. Photoand electropatterning of hydrogel-encapsulated living cell arrays. Lab Chip 2005;5(1):111–118.
66.Bloch J, Fine EG, Bouche N, Zurn AD, Aebischer P. Nerve growth factorand neurotrophin-3-releasing guidance channels promote regeneration of the transected rat dorsal root. Exper Neurol 2001;172(2):425–432.
67.Folch A, Jo BH, Hurtado O, Beebe DJ, Toner M. Microfabricated elastomeric stencils for micropatterning cell cultures. J Biomed Mater Res 2000;52(2):346–353.
68.Ostuni E, Kane R, Chen CS, Ingber DE, Whitesides GM. Patterning mammalian cells using elastomeric membranes. Langmuir 2000;16(20):7811–7819.
69.Gole A, Sastry M. A new method for the generation of patterned protein films by encapsulation in arrays of thermally evaporated lipids. Biotechnol Bioeng 2001;74(2):172– 178.
70.Shiku H, Uchida I, Matsue T. Microfabrication of alkylsilanized glass substrate by electrogenerated hydroxyl radical using scanning electrochemical microscopy. Langmuir 1997;13(26):7239–7244.
71.Turyan I, Matsue T, Mandler D. Patterning and characterization of surfaces with organic and biological molecules by the scanning electrochemical microscope. Anal Chem 2000;72 (15):3431–3435.
72.Amro NA, Xu S, Liu GY. Patterning surfaces using tipdirected displacement and self-assembly. Langmuir 2000; 16(7): 3006–3009.
73.Schwartz PV. Molecular transport from an atomic force microscope tip: A comparative study of dip-pen nanolithography. Langmuir 2002;18(10):4041–4046.
74.Agarwal G, Sowards LA, Naik RR, Stone MO. Dip-pen nanolithography in tapping mode. J Am Chem Soc 2003; 125(2):580–583.
75.Chang JC, Brewer GJ, Wheeler BC. A modified microstamping technique enhances polylysine transfer and neuronal cell patterning. Biomaterials 2003;24:2862–2870.
76.Curtis A, Riehle M. Tissue engineering: the biophysical background. Phys Med Biol 2001; 46(4):R47–R65.
77.Curtis A, Wilkinson C. Reactions of cells to topography. J Biomater Sci Pol Educ 1998;9:1313–1329.
78.Li B, Ma Y, Wang S, Moran PM. A technique for preparing protein gradients on polymeric surfaces: effects on PC12 pheochromocytoma cells. Biomaterials 2005;26:1487– 1495.
79.McFarland CD, Thomas CH, DeFilippis C, Stelle JG, Healy KE. Protein adsorption and cell attachment to patterned surfaces. J Biomed Mater Res 2000;49(2):200–210.
80.Veiseh M, Wickes BT, Castner DG, Zhang MQ. Guided cell patterning on gold-silicon dioxide substrates by surface molecular engineering. Biomaterials 2004;25(16):3315– 3324.
81.Zheng H, Berg MC, Rubner MF, Hammond PT. Controlling cell attachment selectively onto biological polymer-colloid templates using polymer-on-polymer stamping. Langmuir 2004;20(17):7215–7222.
82.Ber S, Kose GT, Hasirci V. Bone tissue engineering on patterned collagen films: An in vitro study. Biomaterials 2005; 16:1977–1986.
83.Dowell-Mesfin NM, Abdul-Karim MA, Turner AM, Schanz S, Craighead HG, Roysam B, Turner JN, Shain W. Topographically modified surfaces affect orientation and growth of hippocampal neurons. J Neural Eng 2004;1(2): 78–90.
84.Mohammed JS, DeCoster MA, McShane MJ. Micropatterning of nanoengineered surfaces to study neuronal cell attachment in vitro. Biomacromolecules 2004;5(5):1745– 1755.
85.Mrksich M, Chen CS, Xia YN, Dike LE, Ingber DE, Whitesides GM. Controlling cell attachment on contoured surfaces with selfassembled monolayers of alkanethiolates on gold. Proc Natl Acad Sci USA 1996;93(20):10775–10778.
86.Theibaud P, Lauer L, Knoll W, Offenhausser A. PDMS device for patterned application of microfluids to neuronal cells arranged by microcontact printing. Biosen Bioelectro 2002; 17:87–93.
87.Scholl M, Sprossler C, Denyer M, Krause M, Nakajima K, Maelicke A, Knoll W, Offenhausser A. Ordered networks of rat hippocampal neurons attached to silicon oxide surfaces. J Neurosci Methods 2000;104(1):65–75.
88.Deutsch J, Motiagh D, Russell B, Desai TA. Fabrication of microtextured membranes for cardiac myocyte attachment and orientation. J Biomed Mater Res 2000;53(3):267– 275.
89.Goessl A, Bowen-Pope DF, Hoffman AS. Control of shape and size of vascular smooth muscle cells in vitro by plasma lithography. J Biomed Mater Res 2001;57(1):15–24.
90.Motlagh D, Senyo SE, Desai TA, Russell B. Microtextured substrata alter gene expression, protein localization and the shape of cardiac myocytes. Biomaterials 2003;24(14):2463– 2476.
91.Zinger O, Zhao G, Schwartz Z, Simpson J, Wieland M, Landolt D, Boyan B. Differential regulation of osteoblasts by substrate microstructural features. Biomaterials 2005;26 (14):1837–1847.
92.Matsuzawa M, Liesi P, Knoll W. Chemically modifying glass surfaces to study substratum-guided neurite outgrowth in culture. J Neurosci Methods 1996;69(2):189–196.
93.Clark P, Britland S, Connolly P. Growth cone guidance and neuron morphology on micropatterned laminin surfaces. J Cell Sci 1993;105:203–212.