
- •VOLUME 1
- •CONTRIBUTOR LIST
- •PREFACE
- •LIST OF ARTICLES
- •ABBREVIATIONS AND ACRONYMS
- •CONVERSION FACTORS AND UNIT SYMBOLS
- •ABLATION.
- •ABSORBABLE BIOMATERIALS.
- •ACRYLIC BONE CEMENT.
- •ACTINOTHERAPY.
- •ADOPTIVE IMMUNOTHERAPY.
- •AFFINITY CHROMATOGRAPHY.
- •ALLOYS, SHAPE MEMORY
- •AMBULATORY MONITORING
- •ANALYTICAL METHODS, AUTOMATED
- •ANALYZER, OXYGEN.
- •ANESTHESIA MACHINES
- •ANESTHESIA MONITORING.
- •ANESTHESIA, COMPUTERS IN
- •ANGER CAMERA
- •ANGIOPLASTY.
- •ANORECTAL MANOMETRY
- •ANTIBODIES, MONOCLONAL.
- •APNEA DETECTION.
- •ARRHYTHMIA, TREATMENT.
- •ARRHYTHMIA ANALYSIS, AUTOMATED
- •ARTERIAL TONOMETRY.
- •ARTIFICIAL BLOOD.
- •ARTIFICIAL HEART.
- •ARTIFICIAL HEART VALVE.
- •ARTIFICIAL HIP JOINTS.
- •ARTIFICIAL LARYNX.
- •ARTIFICIAL PANCREAS.
- •ARTERIES, ELASTIC PROPERTIES OF
- •ASSISTIVE DEVICES FOR THE DISABLED.
- •ATOMIC ABSORPTION SPECTROMETRY.
- •AUDIOMETRY
- •BACTERIAL DETECTION SYSTEMS.
- •BALLOON PUMP.
- •BANKED BLOOD.
- •BAROTRAUMA.
- •BARRIER CONTRACEPTIVE DEVICES.
- •BIOCERAMICS.
- •BIOCOMPATIBILITY OF MATERIALS
- •BIOELECTRODES
- •BIOFEEDBACK
- •BIOHEAT TRANSFER
- •BIOIMPEDANCE IN CARDIOVASCULAR MEDICINE
- •BIOINFORMATICS
- •BIOLOGIC THERAPY.
- •BIOMAGNETISM
- •BIOMATERIALS, ABSORBABLE
- •BIOMATERIALS: AN OVERVIEW
- •BIOMATERIALS: BIOCERAMICS
- •BIOMATERIALS: CARBON
- •BIOMATERIALS CORROSION AND WEAR OF
- •BIOMATERIALS FOR DENTISTRY
- •BIOMATERIALS, POLYMERS
- •BIOMATERIALS, SURFACE PROPERTIES OF
- •BIOMATERIALS, TESTING AND STRUCTURAL PROPERTIES OF
- •BIOMATERIALS: TISSUE-ENGINEERING AND SCAFFOLDS
- •BIOMECHANICS OF EXERCISE FITNESS
- •BIOMECHANICS OF JOINTS.
- •BIOMECHANICS OF SCOLIOSIS.
- •BIOMECHANICS OF SKIN.
- •BIOMECHANICS OF THE HUMAN SPINE.
- •BIOMECHANICS OF TOOTH AND JAW.
- •BIOMEDICAL ENGINEERING EDUCATION
- •BIOSURFACE ENGINEERING
- •BIOSENSORS.
- •BIOTELEMETRY
- •BIRTH CONTROL.
- •BLEEDING, GASTROINTESTINAL.
- •BLADDER DYSFUNCTION, NEUROSTIMULATION OF
- •BLIND AND VISUALLY IMPAIRED, ASSISTIVE TECHNOLOGY FOR
- •BLOOD BANKING.
- •BLOOD CELL COUNTERS.
- •BLOOD COLLECTION AND PROCESSING
- •BLOOD FLOW.
- •BLOOD GAS MEASUREMENTS
- •BLOOD PRESSURE MEASUREMENT
- •BLOOD PRESSURE, AUTOMATIC CONTROL OF
- •BLOOD RHEOLOGY
- •BLOOD, ARTIFICIAL
- •BONDING, ENAMEL.
- •BONE AND TEETH, PROPERTIES OF
- •BONE CEMENT, ACRYLIC
- •BONE DENSITY MEASUREMENT
- •BORON NEUTRON CAPTURE THERAPY
- •BRACHYTHERAPY, HIGH DOSAGE RATE
- •BRACHYTHERAPY, INTRAVASCULAR
- •BRAIN ELECTRICAL ACTIVITY.
- •BURN WOUND COVERINGS.
- •BYPASS, CORONARY.
- •BYPASS, CARDIOPULMONARY.
results of a randomized, double-blind trial. J Thor Cardiovas Sur 2002;124:35–42.
94.Gonzalez P, Hackney AC, Jones S, Strayhorn D, Hoffman EB, Hughes G, Jacobs EE, Orringer EP. A phase I/II study of polymerized bovine hemoglobin in adult patients with sickle cell disease not in crisis at the time of study. J Investig Med 1997;45:258–264.
95.Feola M, Simoni J, Angelillo R, Luhruma Z, Kabakele M, Manzombi M, Kaluila M. Clinical trial of a hemoglobin based blood substitute in patients with sickle cell anemia. Surg Gynecol Obs 1992;174:379–386.
96.Mullon J, Giacoppe G, Clagett C, McCune D, Dillard T. Transfusions of polymerized bovine hemoglobin in a patient with severe autoimmune hemolytic anemia. N Engl J Med 2000;342:1638–1643.
97.Sloan EP. The clinical trials of diaspirin cross-linked hemoglobin (DCLHB) in severe traumatic hemorrhagic shock: the tale of two continents. Int Care Med 2003;29: 347–349.
98.Gould SA, Moore EE, Hoyt DB, Ness PM, Norris EJ, Carson JL, Hides GA, Freeman IH, DeWoskin R, Moss GS. The lifesustaining capacity of human polymerized hemoglobin when red cells might be unavailable. J Am Coll Surg 195: 445–452; discussion 2002; 452–455.
99.Lieberthal W, Fuhro R, Freedman JE, Toolan G, Loscalzo J, Valeri CR. O-raffinose cross-linking markedly reduces systemic and renal vasoconstrictor effects of unmodified human hemoglobin. J Pharmacol Exper Therap 1999;288:1278–1287.
100.Cheng DC, Mazer CD, Martineau R, Ralph-Edwards A, Karski J, Robblee J, Finegan B, Hall RI, Latimer R, Vuylsteke A. A phase ii dose-response study of hemoglobin raffimer (Hemolink) in elective coronary artery bypass surgery. J Thor Cardiov Sur 2004;127:79–86.
101.Greenburg AG, Kim HW, Hemolink Study Group. Use of an oxygen therapeutic as an adjunct to intraoperative autologous donation to reduce transfusion requirements in patients undergoing coronary artery bypass graft surgery. J Am Coll Surg 198:373–383; discussion 2004; 384–385.
102.Conover CD, Lejeune L, Shum K, Gilbert C, Shorr RG. Physiological effect of polyethylene glycol conjugation on stroma-free bovine hemoglobin in the conscious dog after partial exchange transfusion. Artif Organs 1997;21:369– 378.
103.Teicher BA, Ara G, Herbst R, Takeuchi H, Keyes S, Northey D. Peg-hemoglobin: effects on tumor oxygenation and response to chemotherapy. In Vivo 1997;11:301–311.
104.Linberg R, Conover CD, Shum KL, Shorr RG. Increased tissue oxygenation and enhanced radiation sensitivity of solid tumors in rodents following polyethylene glycol conjugated bovine hemoglobin administration. In Vivo 1998;12: 167–173.
105.Serna DL, Powell LL, Kahwaji C, Wallace WC, West J, Cogert G, Smulowitz P, Steward E, Purdy RE, Milliken JC. Cardiac function after eight hour storage by using polyethylene glycol hemoglobin versus crystalloid perfusion. ASAIO J 2000;46:547–552.
106.Meng FT, Zhang WZ, Ma GH, Su ZG. The preparation and characterization of monomethoxypoly(ethylene glycol)-b- poly-dl-lactide microcapsules containing bovine hemoglobin. Artf Cells, Blood Sub Immobil Biotechnol 2003;31:279– 292.
107.Phillips WT, Klipper RW, Awasthi VD, Rudolph AS, Cliff R, Kwasiborski V, Goins BA. Polyethylene glycol-modified liposome-encapsulated hemoglobin: a long circulating red cell substitute. J Pharmacol Exp Ther 1999;288:665–670.
See also BIOCOMPATIBILITY OF MATERIALS; BLOOD COLLECTION AND PROCESSING; BLOOD GAS MEASUREMENTS.
BONE AND TEETH, PROPERTIES OF |
523 |
BONDING, ENAMEL. See RESIN-BASED COMPOSITES.
BONE AND TEETH, PROPERTIES OF
RODERIC LAKES
University of Wisconsin
Madison, Wisconsin
J. LAWRENCE KATZ
University of Missouri
Kansas City, Missouri
INTRODUCTION
Bone has a variety of functions in the body of which some of the most important are structural in nature: protection of vulnerable body parts, support of the body, and to provide muscle attachments. A knowledge of the mechanical and adaptive properties of bone is useful in the design and use of prostheses that replace a bone or a portion of a bone. Mechanical properties of bone are also of interest in trauma biomechanics and in efforts to prevent injury to the body. As for teeth, they also are replaced by artificial materials which are called upon to perform the mechanical functions of the original tooth. This article contains a survey of known properties of bone and teeth and their components collagen and apatite, with an emphasis on bone and its mechanical properties.
A voluminous literature is available dealing with the properties of bone and to a lesser extent of teeth’s major constituents: collagen and apatite. Reported properties are often found to differ. Some of the differences arise from the fact that bone and tooth structures are of biological origin and consequently vary depending on the individual and on the part of the body from which the specimen is taken. Other differences are due to experimental technique and variations in environmental conditions during experiments. Of necessity, results presented in this article are selected from a large mass of published reports. The authors have endeavored to select results obtained by good techniques and representative of accepted values. Nevertheless, other results obtained by equally good techniques may be expected to differ somewhat as a result of biological variability. Therefore, it is suggested that additional measurements of the properties of bone and teeth can be found in several of the source books listed in the Bibliography [see (1–10)].
MECHANICAL PROPERTIES OF COMPACT BONE
Compact Bone Structure
The mechanical properties of bone are inseparably related to its structure. Bone tissue is a complex composite material that at different levels of scale exhibits fibrous, porous, and particulate microstructural features (1–5). The following constituents are present in bone: mineral, protein, other organic materials, and fluids such as water. The mineral, principally a carbonated apatite, where the

524 BONE AND TEETH, PROPERTIES OF
carbonate group substitutes in part for the phosphate group [Ca10(PO4,CO3)6(OH)2] (5,6). In mature bovine cortical bone (similar to human cortical bone) it occurs as microcrystalline inclusions of plate-like shape (mineralites) of dimensions 0.7 11 17 nm (11). However, in young postnatal bovine bone, the mineralites are thicker, shorter, and narrower, [i.e. 2 6 9 nm (12)]. These mineralite sizes measured by AFM are closest to the actual values as, ‘‘AFM yields the full three-dimensional structure of mineralites rather than a projection ’’ thus providing the full shape of each mineralite measured. The other major techniques for measuring mineralite sizes, transmission electron microscopy (TEM) and X-ray diffraction line broadening, each suffer from artifacts that result in increased sizes of some of the dimensions (3).
On the ultrastructural level (nanoscale), the mineral crystallites are in intimate apposition with fibrils of the protein collagen. A, ‘‘(d)iagrammatic depiction of the supramolecular packing of collagen molecules in a fibril ’’ plus the possible arrangement of the mineralites within a fibril is given as Fig. 7 in Ref. 12. These fibrils are from 20 to 200 nm in diameter and are organized into fibers that are in turn arranged in bone into lamellae or layers. The fibers in each lamella run longitudinally, spirally, or nearly circumferentially. Moreover, the orientation of these layers are different in alternate lamellae. A micromechanical model for the Young’s modulus of bone has been proposed, based on these histological features, however, it has not yet been tested experimentally. The organization of collagen fibrils differs in woven bone and lamellar bone. Mineralized collagen fibrils and isolated crystals from the mid-dia- physes of human fetal femurs were observed with scanning, TEM, and high resolution electron microscopy. The apatite crystals in woven bone are also platelet shaped, similar to mature crystals from lamellar bone. Average crystal dimensions are considerably smaller in woven bone than those of mature crystals in lamellar bone. In diseased bone such as that affected by osteogenesis imperfecta, the apatitic crystals occur in various sizes and shapes; they are oriented and aligned with respect to collagen in a manner that differs from that found in normal calcified tissues.
In compact cortical bone, the lamellae are layers arranged circumferentially around a central canal and form the Haversian system (secondary osteon). Haversian canals typically contain small blood vessels. Haversian bone occurs in the cortices of bones in adult humans (1– 3,5) and in the bones of various large animals (1,2). Osteons are roughly cylindrical structures up to 200 mm in diameter. In a long bone, they tend to run approximately parallel to each other and to the bone axis. Figure 1 displays the typical structure of human cortical bone, whereas Fig. 2 displays the typical structure of human cancellous (also known as trabecular or spongy) bone. The large circular or elliptical features in the former figure are the cross-sections of osteons, the concentric layers are lamellae, and the central dark circles are the cross-sections of Haversian canals. The numerous spots among the lamellae are the lacunae, in which the osteocytes, or bone cells live. The lacunae are roughly ellipsoidal and have dimensions of10 15 25 mm. The osteocytes have many thin processes that occupy channels in the bone matrix known as
Figure 1. Human Haversian bone. Reflected light micrograph of a specimen cut perpendicular to the bone axis. Scale mark: 200 mm.
canaliculi; they are too small to be visible in Fig. 1. The mechanical properties of bone depend on its degree of bulk and surface hydration and the details of its structure that depend on variables such as the age, state of health, and level of physical activity of the individual, the location of the bone in the body, as well as the rate and direction at which load is applied to the bone.
The emphasis in this article is on wet skeletal tissues, bone and teeth, from healthy adults, with occasional references to dry tissues for comparison. However, there are many studies of mammalian skeletal tissues as they provide a useful counterpart to the human studies; studies of the properties of bovine bone and teeth are the most numerous in this respect. The structures of both mature bovine cortical and cancellous bone are very similar to those corresponding human tissues Figs. 1 and 2, respectively. Young bovine cortical bone structure is quite different as seen in Fig. 3. This plexiform (or lamellar) bone resorbs and remodels to Haversian bone as the animal matures. Comparison of the young and mature bovine bone properties provides added insight into the importance of structure in determining the mechanical properties of bone. Thus, where appropriate, properties of bovine bone and teeth are included in Tables 1–7.
Figure 2. Human cancellous bone from the proximal femur. The marrow has been removed. Scale mark: 5 mm.

BONE AND TEETH, PROPERTIES OF |
525 |
Figure 3. Bovine plexiform bone. Reflected light micrograph of a specimen cut perpendicular to the bone axis. Scale mark: 200 mm.
Elasticity of Compact Bone: Comparison with Teeth and Other Materials
Elastic materials deform under load; elasticity entails reversible behavior in which the material returns to its original configuration after the load is removed. If the load is sufficiently small, there is a linear relationship between stress and strain. For simple tension or compression, the constant of proportionality is referred to as Young’s modulus E and for shear or torsion it is the shear modulus G(4,29). The stiffness of human compact bone as quantified by Young’s modulus typically lies between 12 and 25 GPa for tension or compression depending on sample orientation and experimental technique (17), (1 GPa ¼ 145,000 lb in. 2). It has been suggested that the tensile and compressive elastic moduli are not equal, but at small strain, the best evidence indicates that tensile and compressive properties are identical (13).
In Table 1, some examples of the elastic moduli of wet human bone and teeth are compared with those of various other materials. The mechanical testing strain rates for bone are faster than those encountered by bones in walking, but are perhaps comparable to those in vigorous activities. They are slower than those that occur during
Table 1. Bone, Teeth, and Other Materials: Stiffness
fracture. Compact bone is 10 times stiffer than most ‘‘rigid’’ polymers, but is about one-tenth as stiff as common metals such as steel. Table 1 also shows the relationship between stiffness and density. This relationship governs the structural efficiency of whole bones. In view of the fact that the skeleton represents 17% of the weight of the human body, structural efficiency of bones is relevant to their performance in the body. For example, the overall rigidity per unit weight of a bone acting as a short column or as a tensile member is proportional to the modulus to density ratio, E/r, of the bone tissue of which it is made. By contrast, for a given weight of material, the Euler buckling load of a bone acting as a slender column or the bending stiffness of a bone acting as a beam of constant shape is proportional to E/r2(2,6). Density enters these relations in a different way since the rigidity of a short column depends on its cross-sectional area while the bending rigidity of a beam is governed by its moment of inertia.
A more complete listing of the properties of both human and bovine bone and teeth is given in Table 2, the latter data are included because of the similarity in hierarchical structure and organization of mature bovine compact bone with that of human compact bone. These data were obtained using various techniques, mechanical testing, ultrasonic wave propagation (UWP). In the low megahertz (MHz) region (2–5 MHz), scanning acoustic microscopy (SAM) in the high megahertz region (400–600 MHz), and nanoindentation. The first three techniques also were used to obtain the teeth data. Here too, the properties are strongly dependent on the sample location and orientation, for example, the range in dentin properties over the sample surface obtained by SAM (21), Table 2. Corresponding properties of human trabecular bone volumes and individual trabeculae are given in Table 7.
Because SAM is not as well documented compared to either UWP or mechanical testing (MT) in obtaining elastic properties, a description of the technique follows below.
Scanning Acoustic Microscopy
The development of SAM (30,31); see also (9) enabled the analysis of the biomechanical properties of materials at much higher resolution than was previously achieved using traditional ultrasonic wave propagation techniques.
Material |
Young’s Modulus E, GPa |
Density (r), g cm 3 |
E/r |
E/r2 |
Human compact bone |
|
|
|
|
longitudinal direction (13) |
17 |
1.8 |
9.4 |
5.2 |
transverse direction |
12.5 |
|
|
|
Tooth dentin (7,14) |
18 |
2.1 |
8.6 |
4.1 |
Tooth enamel (7,15) |
50 |
2.9 |
17 |
6.0 |
Polyethylene (high density) (4) |
0.5 |
0.95 |
0.53 |
0.55 |
Polymethyl methacrylate (4) |
3.0 |
1.2 |
2.5 |
2.2 |
Steel(structural) |
200 |
7.9 |
25 |
3.2 |
Aluminum |
70 |
2.7 |
26 |
9.5 |
Granite |
70 |
2.8 |
25 |
9.1 |
Concrete |
25 |
2.3 |
11 |
4.6 |
Wood(pine) |
11 |
0.6 |
18 |
30 |
|
|
|
|
|
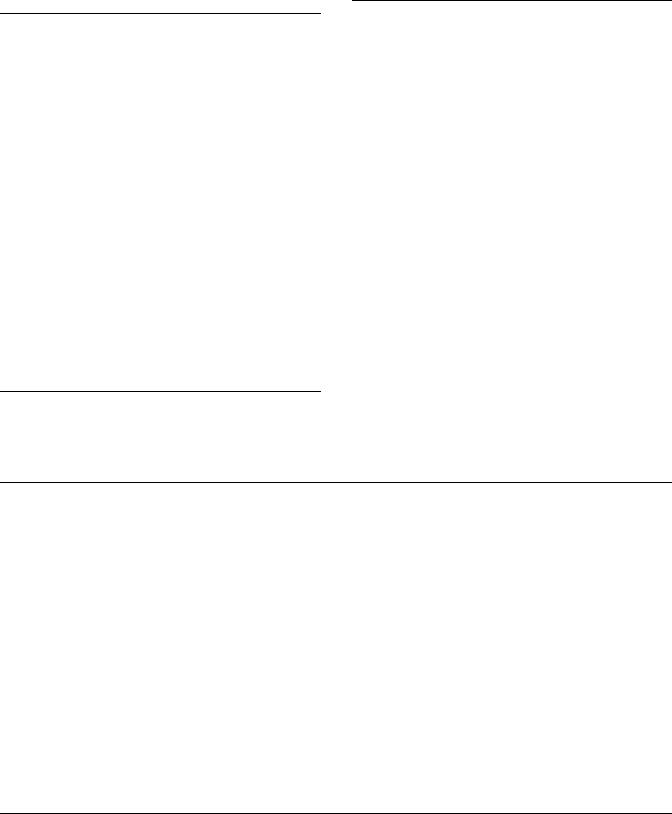
526 BONE AND TEETH, PROPERTIES OF
Table 2. Elastic Properties of Wet Human and Bovine Bone and Teeth
Material |
Young’s Modulus, GPa |
References |
|
|
|
Human compact bone |
|
|
axial direction (femur) |
27.7 (U)a |
16 |
|
17.6 (M)a |
17 |
|
23.4 (S)a |
18 |
(osteons) |
22.4 (N)a |
19 |
(interstitial lamellae) |
25.7 (N) |
19 |
radial direction (femur) |
18.9 (U) |
16 |
|
12.5 (M) |
17 |
|
13.0 (N) |
19 |
Human teeth |
|
|
dentin |
13.0 (S) |
20 |
|
16.4–38.6 (S) |
21 |
enamel |
62.7 (S) |
20 |
Bovine compact bone |
|
|
axial direction (tibia) |
36.0 (M) |
2 |
axial direction (femur) |
22.7 (M) |
17 |
axial direction (femur) |
21.9 (U) |
22 |
radial direction (tibia) |
22.8 (M) |
2 |
radial direction (femur) |
10.3 (M) |
17 |
radial direction (femur, |
13.1 (U) |
22 |
average) |
|
|
Bovine teeth |
|
|
dentin |
26.3 (U) |
23,24 |
dentin |
25.2 (U)b |
25 |
enamel |
105.1 (U) |
23,24 |
enamel |
97.8 (U)b |
25 |
aU ¼ Ultrasonics; M ¼ Mechanical Testing; S ¼ Scanning Acoustic Microscopy; N ¼ Nanoindentation.
bAverage of measurements of several samples.
Table 5. Comparison of Materials: Tensile Strength
|
Strength |
Density |
|
Material |
sult, MPa |
r, g cm 3 |
sult/r |
Human femoral compact |
|
|
|
bone (17), |
|
|
|
longitudinal direction |
148 |
2.0 |
74 |
transverse direction |
49 |
2.0 |
25 |
Bovine femoral plexiform |
|
|
|
bone, (13) |
|
|
|
longitudinal direction |
167 |
2.0 |
83 |
Tooth dentin (8), average |
271 |
2.1 |
130 |
Tooth enamel (8), average |
275 |
2.9 |
95 |
Polyethylene (high density) |
20–40 |
0.95 |
21–42 |
Poly(methyl methacrylate) |
70 |
1.2 |
59 |
(PMMA) |
|
|
|
Steel(structural) |
400 |
7.8 |
51 |
Aluminum(1100-H14) |
110 |
2.7 |
41 |
Granite |
20 |
2.8 |
7.2 |
Concrete(compression) |
28 |
2.3 |
12 |
|
|
|
|
A significant advantage of SAM is the ability to investigate the properties of internal and subsurface structures in addition to the surface properties of most materials, including those that are optically opaque. Another advantage of special interest for studying biological materials is that a liquid couplant must be used to transmit the acoustic waves from the acoustic lens to the specimen being studied, thus the specimen is kept wet during all measurements. Therefore, fresh tissue specimens can be used as well as embedded specimens. In addition, the use of high quality,
Table 3. Elastic Anisotropy of Bovine and Human Bone
|
Tensorial Elastic Moduli (GPa), Determined Ultrasonically Wet |
|
|
|
||||||
|
|
|
Bovine Femur (26) |
|
Dry Human Femur (27) |
|||||
|
|
|
|
|
|
|
|
|
||
Elastic constant |
Haversian (transverse isotropic) |
Plexiform (orthotropic) |
Haversian (transverse isotropic) |
|||||||
|
|
|
|
|
|
|
|
|
|
|
C11 |
21.2 |
|
|
22.4 |
|
|
|
|
23.4 |
|
C22 |
21.0 |
|
|
25.0 |
|
|
|
|
|
|
C33 |
29.0 |
|
|
35.0 |
|
|
|
|
32.5 |
|
C44 |
6.30 |
|
|
8.20 |
|
|
|
|
8.71 |
|
C55 |
6.30 |
|
|
7.10 |
|
|
|
|
|
|
C66 |
5.40 |
|
|
6.10 |
|
|
|
|
|
|
C12 |
11.7 |
|
|
14.0 |
|
|
|
|
9.06 |
|
C13 |
12.7 |
|
|
15.8 |
|
|
|
|
|
|
C23 |
11.1 |
|
|
13.6 |
|
|
|
|
9.11 |
|
Table 4. Elastic Anisotropy of Bonea |
|
|
|
|
|
|
|
|
||
Young’s Moduli, GPa |
|
Shear Moduli, GPa |
|
Poisson’s Ratios, Dimensionless |
||||||
|
|
|
|
|
|
|
|
|
||
Human |
Bovine |
|
Human |
Bovine |
Human |
Bovine |
||||
|
|
|
|
|
|
|
|
|
|
|
b |
|
|
|
G ¼ 3.6 |
|
|
|
v ¼ 0.58 |
|
|
E ¼ 17.0c |
22 |
|
|
5.3 |
|
|
0.30 |
|||
E ¼ 11.5d |
15 |
|
|
G ¼ 3.3 |
6.3 |
|
|
v ¼ 0.31 |
0.11 |
|
E ¼ 11.5 |
12 |
|
|
G ¼ 3.3 |
7.0 |
|
|
v ¼ 0.31 |
0.21 |
aTechnical elastic moduli. Wet human femoral bone by mechanical testing (13) and bovine femoral bone by ultrasound (23). bRadial direction.
cCircumferential direction. dLongitudinal direction.
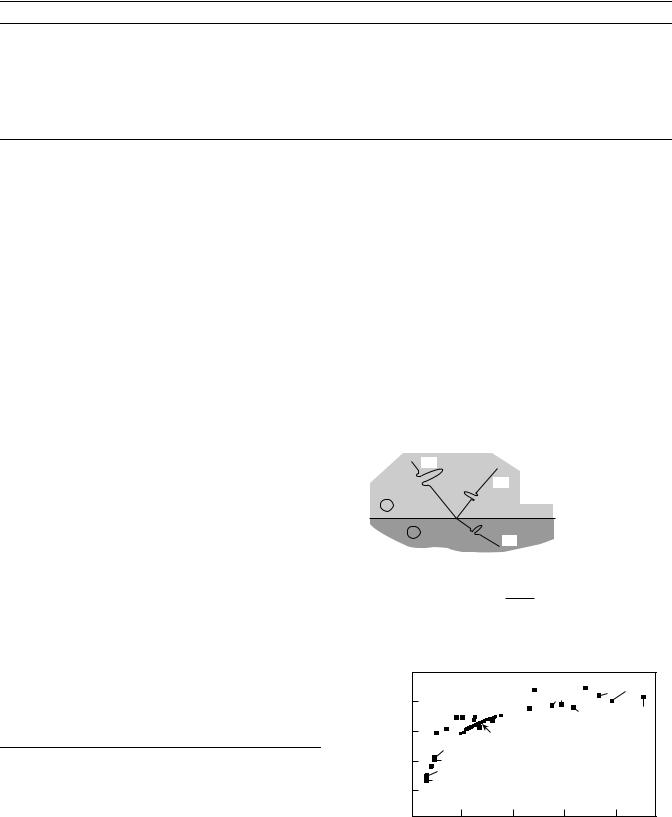
Table 6. Elastic Properties of Apatites
Material |
Young’s modulus, GPa |
Hydroxyapatite |
|
(polycrystalline) |
117 |
(single crystal, modelinga) |
120 |
Fluoroapatite |
|
(polycrystalline) |
120 |
(single crystal, ultrasonicsa) |
130 |
Chloroapatite |
|
(polycrystalline) |
94.3 |
aCalculated from single-crystal elastic constants.
high frequency focusing acoustic lenses permits examination of the elastic properties of biological materials on a microscope scale comparable to the optical histology studies. The heart of the SAM is a spherical lens formed at the interface between a high acoustic velocity solid (e.g., sapphire) and the low acoustic velocity couplant liquid. Due to the high acoustic ‘‘refractive index’’, spherical aberration is negligible and an acoustic beam displaying significant convergence can be obtained. A radio frequency (RF) signal is generated from the transmitter. The acoustic lens is equipped with a piezoelectric transducer that converts the RF signal into an acoustic wave. This signal is then made to converge by the lens and propagates to the specimen through the coupling liquid. When reaching the surface of the specimen, a part of the acoustic wave is reflected back through the lens to the transducer, that, acting now as a receiver, transforms the acoustic signal into an RF signal. The amplitude of the echo reflected back to the lens is a measure of the acoustic reflectivity of the surface of the investigated material at the point in focus. It is proportional to the reflection coefficient, r, which is related to the acoustic impedances of the liquid couplant, Z1, and the investigated material, Z2, by the equation r given in Fig. 4. Acoustic impedance, Z, is measured in Rayls and is defined as Z ¼ rv, where r is the material density and v is the velocity of the longitudinal (dilatational) acoustic wave propagating in the direction perpendicular to the surface. The images present the variations in acoustic signals that originate either from the intrinsic acoustic reflectivity of the material surface or through interference occurring between different surface and subsurface reflected signals. In the former case, surface imaging, the acoustic reflectivity variations are a result of the local changes in acoustic impedance (32).
Table 7. Elastic Modulus of Trabecular Bone Volumes
and Trabeculae
Trabeculae |
Elastic Modulus, GPa |
Reference |
|
|
|
Human trabecular bone |
17.4 (S) |
18 |
(Trabeculae) |
|
|
(longitudinal) |
19.4 (N) |
19 |
(transverse) |
15.0 (N) |
19 |
Human trabecular |
|
|
bone volumes |
|
|
(proximal tibia) |
0.445 (M) |
3 |
|
|
|
|
BONE AND TEETH, PROPERTIES OF |
527 |
Bulk modulus, GPa |
Shear modulus, GPa |
Reference |
88.0 |
45.5 |
27 |
111 |
45.3 |
8 |
94.0 |
46.4 |
27 |
117 |
49.4 |
28 |
68.5 |
37.1 |
27 |
Figure 5 is a plot of elastic stiffness (GPa) versus reflection coefficient, r, for a wide range of materials. Bone has an acoustic impedance in the neighborhood of Z ¼ 7.5 Mrayls, yielding a reflection coefficient in the neighborhood of r ¼ 0.67. Of course, Z for bone can vary over a considerable range depending on a number of factors that would affect both the density and structural cohesivity of the specific specimen being measured. Likewise, Z for water will vary depending on the temperature at which the couplant fluid is maintained during the experiment, for example, at 0 8C, Z(H2O) ¼ 1.40 Mrayl; at body temperature, 37 8C, Z(H2O) ¼ 1.51 Mrayl; and at 60 8C, Z(H2O) ¼ 1.53, due mainly to the variations in water’s acoustic properties with temperature.
As described above, the high frequency mode at 400 and 600 MHz also has been used to study the in vitro micromechanical elastic properties of human trabecular and
I |
r : Density |
R |
v : Long velocity |
r : Reflection coefficient |
|
1 |
z : Acoustic Impedance |
|
|
2 |
z 1 = r1v 1 |
z 2 = r2v 2 |
|
T |
|
r = Ar/A1
r= z2−z1 z2+z1
Figure 4. Schematic diagram of the incident, reflected, and transmitted signals at the interface between two materials.
R( ) |
|
|
B1 |
Cd |
Silica |
−8 |
|
|
Glass |
|
|
|
|
|
|
||
coefficient |
Nylon |
|
Mg |
Quartz |
|
|
|
C |
Brick |
||
|
−6 |
|
|
|
|
|
Epon |
Bone |
|
|
|
|
|
|
|
||
Reflection |
−4 |
Lucite |
|
|
|
|
Epoxy |
|
|
|
|
|
−2 |
Polystyrene |
|
|
|
|
|
|
|
|
20 |
40 |
60 |
80 |
Elastic stiffness (GPA)
Figure 5. Reflection coefficient, r versus Modulus, E.

528 BONE AND TEETH, PROPERTIES OF
Figure 6. (a) The 400 MHz SAM image of two hypomineralized osteons from human femoral cortical bone. (b) Same area as in part a showing the line along which an x–z interference image was taken. (c) The x–z Interference image taken along the line shown in part b.
compact cortical femoral bone (15,31). In this study, they were able to image the properties of the individual osteonic lamellae at high enough resolution so that three new micromechanical observations were made: (1) the outermost lamellae, always appear to be more compliant; (2) the outermost lamellae of adjacent abutting osteons appear to have the same acoustic impedance (and thus Young’s modulus), even though structurally distinct; and (3) adjacent lamellae within an osteon alternate in their acoustic impedance (and thus Young’s modulus).
Scanning acoustic microscopy is particularly powerful in providing physical evidence of the possible differences in sound velocity in regions of significant differences in gray level, that is, the darker the gray level, the lower the acoustic impedance, the brighter the gray level, the higher the acoustic impedance, Fig. 6a–c. Figure 6a is a SAM micrograph (400 MHz Burst mode, 1208 aperture lens) of human femoral cortical bone (18). The two much darker Haversian systems in the middle of the image are surrounded by the types of Haversian systems (secondary osteons) usually observed in normal bone. The startling difference in gray levels could arise due to out-of-focus artifacts developed in cutting or polishing the specimen. However, performing a x–z curve along the line depicted in Fig. 6b, which goes through the lower, darker Haversian
system and the surrounding tissues, yields the image, Fig. 6c; note that the upper level of the broad band is essentially level, indicating that the specimen surface is everywhere level and normal to the acoustic beam. More important, the narrow band and secondary reflection corresponding to the dark Haversian reflects the lower Z and r for the Haversian. A possible explanation for the Haversians is that there was an arrested state of development leading possibly to a hypomineralized area. Unfortunately, we do not have information concerning the possibility of a disease state or a drug modality responsible for these two underdeveloped Haversians that possibly formed just prior to the death of the individual. They are illustrated here to show the ability of the SAM to permit highly sensitive measurements, at high resolution, of variations in Z and r due to remodeling.
In order to convert from reflection coefficient to Young’s modulus on the SAM scans of materials of unknown properties, taken with the Olympus UH3 SAM, it is necessary to develop three calibration curves—voltage (V) versus reflection coefficient (r); reflection coefficient (r) versus acoustic impedance (Z); and acoustic impedance (Z) versus Young’s modulus (E)—based on using the values of known materials ranging from polymers at the low end of r to metals and ceramics at the high end (18).
The stiffness of compact bone tissue depends on the bone from which it is taken. Fibular bone has a Young’s modulus18% greater, and tibial bone 7% greater than that of femoral bone (33). The differences are associated with differences in the histology of the bone tissue. Femoral bone, for example, has a high proportion of osteons that appear light in the polarizing microscope and that have a preponderantly circumferential collagen fiber orientation (34). The histology of a bone is unquestionably related to its function in the body. The relationship has been explored in some detail in the context of bones of very different function in various species of animals (1,2).
Bone is elastically anisotropic, that is, its properties depend on direction. Such behavior is unlike that of steel, aluminum, and most plastics, but is similar to that of wood. Anisotropic properties of bone are shown in Tables 3 and 4. As can be seen from the data in Table 4, human compact femoral bone is 1.5 times as stiff in the longitudinal direction as it is in the transverse directions. The shear modulus G, determined from a torsion test upon a specimen aligned with the bone axis, is 3.3 GPa (13), so that E/ G ¼ 5.15. Such a small value of G in comparison with Young’s modulus E is a further manifestation of the anisotropy of cortical bone. For normal isotropic materials (positive Poisson’s ratio), E/G, must lie between 2 and 3 and is typically 2.6. Detailed studies of the anisotropy of bone have been conducted using ultrasonic methods as well as by mechanical testing. The elastic constants given in Table 3 are components of the elastic modulus tensor Cijkl in the following relation between stress sij and strain ekl in an anisotropic material. The 3 axis is here assumed to be the bone’s longitudinal axis, the 2 direction is circumferential, and the 1 direction is radial.
X3 X3
sij ¼ Cijklekl k¼1 l¼1
In this equation, indexes i and j can have values 1, 2, or 3, so that there are nine components of stress of which six are independent. Since there are six independent components of strain, the number of independent C values is reduced from 81 to 36. Consideration of conservation of mechanical energy further reduces the number of elastic constants to 21, for the least symmetric anisotropic material (7). Materials that have some structural symmetry are described by fewer anisotropic elastic constants. For example, an orthotropic material, (e.g., wood), with three perpendicular planes of symmetry is described by nine constants. A material with transverse isotropic symmetry, (i.e., one that appears the same under an arbirary rotation about an axis), is described by five constants. In crystal physics, the former symmetry is referred to as orthotropic, while the latter is referred to as hexagonal. An isotropic material appears the same under any rotation and has the same properties in any direction. Two independent elastic constants are needed for the description of the elastic behavior of such a material. Equation 1 can be reduced to a more tractable form by a compaction of the counting indices, the so-called Einstein notation, that is, si ¼ Cijej, where 11 ! 1, 22 ! 2, 33 ! 3, 23 ! 4, 13 ! 5, 12 ! 6 (e.g., C1123 ! C14). This reduced notation is used in Table 3.
BONE AND TEETH, PROPERTIES OF |
529 |
Both structural considerations and experiments indicate that human compact bone has five independent elastic constants and therefore exhibits transverse isotropic symmetry (27,35,36). Results of a different ultrasonic experiment suggest different stiffnesses in the radial and circumferential directions (16). Based on these results, it has been proposed that human compact bone is orthotropic. The difference between the stiffnesses in the radial and circumferential direction is, however, small and has been attributed to the gradient in porosity going from the periostium to the endostium. Thus, for modeling purposes transverse isotropy is the appropriate choice of symmetry (37,38).
Note that the elastic moduli found at high frequencies by ultrasonic methods are greater than those obtained statically or at low frequencies via mechanical testing machines. This is a result of the rate dependence (viscoelasticity) of bone. In the mechanics of whole bones, the principal macroscopic manifestation of cortical bone tissue anisotropy is that the bending rigidity of a bone (the femur) is much greater than its torsion rigidity (39). The degree of anisotropy and the symmetry of bone tissue depends on the species and on the location of the bone in the body. Bovine plexiform bone is stiffer than human Haversian bone, but dry bone is stiffer than the same type of bone when wet. Bovine plexiform bone is orthotropic and has significantly different elastic moduli in the longitudinal, radial, and circumferential directions (40). These properties reflect the laminar architecture of this type of bone, as shown in cross-section in Fig. 2. The scale mark is in the radial direction and is perpendicular to the laminae. Bovine plexiform bone is a type of primary bone that occurs in relativley young cattle. It is often used for experiments as a result of its availability. Canine femoral bone is also orthotropic (16), however, canine mandibular bone and possibly also human mandibular bone, is transversely isotropic (41).
Anisotropic properties of bone may also be expressed in terms of the technical elastic constants, Young’s modulus E, shear modulus G, and Poisson’s ratio, v, for different directions. Poisson’s ratio is minus the transverse strain divided by the axial strain in the direction of stretching or of compressing force. Representative values for dry human femoral bone are shown in Table 3. The 3 direction is the long axis of the bone, the 2 direction is circumferential, and the 1 direction is radial. We note that Poisson’s ratio in isotropic materials must be less than one-half (7). In anisotropic materials, larger values are permissible, so that the values reported for bone do not violate any physical law.
Bone mineral density plays an important role in determining all of the elastic properties described above. Under normal physiological and physical conditions, as bone density increases so do the respective elastic properties. However, bone density alone does not always determine fracture risk in pathologies such as osteporosis. A general term in vogue now, ‘bone quality’, is being used to qualify what is information is necessary to determine when bone is at risk of failure due to reduced density. Structure–property relationships are the key here, especially in understanding when trabecular bone will fail. Even under the conditions of reduced density, the appropropriate structural organization
530 BONE AND TEETH, PROPERTIES OF
of the bone may still provide adequate support during normal function. Similarly, good density alone, if associated with a genetic pathology, such as found in osteopetrosis, will not provide adequate prrotection against fracture. The hardness and elastic moduli of osteopetrotic cortical bone are even well below that of osteoporotic bone even though the density of the former is in the normal range (26,42). Indeed, osteopetrotic bone tends to fracture quite readily.
It is clear that for a detailed modeling of the elastic properties of bone, its complex hierarchical structure must be taken into account (37,38,43).
Strength
The ultimate strength of bone tissue refers to the maximum stress the material can withstand before breaking. The tensile strength of human compact bone (17) in a direction parallel to the osteons is about 150 MPa or 21,000 lb in. 2 (1 MPa ¼ 145 lb in. 2). As indicated in Table 5, bone is stronger than various plastics, concrete, brick, some metals, (e.g., aluminum), and most woods. Although bone is stronger than aluminum, commonly used aluminum alloys are stronger than bone. Even so, bone has a lower density than aluminum and a much lower density than steel. The criterion for structural strength in beam bending is material strength divided by the 1.5 power of density [3. ]. For bending of plate-shaped structural elements the criterion is material strength divided by the square of the density. The strength to density ratio for bone is greater than that for structural steel. Therefore bone has very favorable properties in comparison with steel and is competitive with aluminum alloys. Bone is anisotropic in its strength as well as in its elastic behavior. In particular, bone is considerably weaker when loaded transversely than when loaded along the osteon direction. Fortunately, bone in the body does not normally experience significant transverse loads. Several investigators have explored the dependence of bone strength upon age. Tensile strength of adult compact bone decreases 4% per decade of age (44) as does its shear strength (45). In other studies, no significant age dependence of strength was found (46,47). More recently, it was found that the tensile strength of femoral bone decreases 2.1% per decade of age while that of tibial bone decreases 1.2% per decade of age (48). The decrease in strength was statistically significant in the case of femoral bone, but not in the case of tibial bone. Both kinds of bone exhibit significant decreases, 6.8% per decade for femur and 8.4% per decade for tibia, in energy absorption to fracture, a measure of toughness. No significant difference between age-matched males and females was found for any mechanical property (48). Tibial bone is stronger in tension than femoral bone by 19% (49,50) and is 4% stronger than fibular bone.
Currey observes that the average stiffness of human and sheep bone increases monotonically with age (in humans from age 2–50), while energy absorption to fracture decreases. The lower mineralization and stiffness and higher toughness seen in young bones is considered adaptive in view of the many falls and bumps experienced by children and young animals (1).
The fracture behavior of a material is not described fully by its yield and ultimate strengths alone; toughness is also
important. Toughness is seen as an important characteristic of bone in view of the microcracks that occur in living bone. Fracture of laboratory specimens containing controlled notches provides information concerning the toughness of materials (49,50). For example, the nominal
fracture strength of a tensile specimen with an edge notch of length a is sult ¼ K1ca 1/2/Y in which Y depends on the specimen geometry and K1c is called the critical stress
intensity factor. A large value of K1c results in high strength even in the presence of a notch; K1c is a measure of material toughness.
The critical stress intensity factor K1c for fracture of
compact tension specimens of bovine femur bone is from 2.2 to 4.5 MN m 3/2, and the specific surface energy for frac-
ture is from 390 to 560 J m 2 (49). The toughness does not, however depend on the sharpness of the controlled notch in the expected way; the significance of this observation is discussed in the section Compact Bone as a Composite Material. As for the age dependence of bone fracture toughness, the energy absorbed to fracture is observed to decrease during childhood and early middle age, and the elastic modulus increases (51). Recently, fracture surfaces from accident victims have been examined microscopically (52). Such surfaces are observed to be much rougher and more intricate than fracture surfaces produced in laboratory specimens of dead bone, which suggests a greater toughness in living bone. The influence of bone viability on its mechanical properties is not well understood. The dependence of bone elastic modulus on viability has been explored in several studies, but the evidence for a difference in elasticity is not compelling.
Currey (1), compares density, modulus, strength and toughness of bones from deer antler, cow thigh, and whale tympanic bulla. As one might expect, modulus increases and toughness decreases with density. Strength is the highest for the femoral bone. Properties vary considerably between these types of bone; the difference is attributed largely to mineralization but partly to histology. The high mineralization of the whale ear bone is responsible for its stiffness, density and brittleness. The stiffness and density are useful given the acoustic function of the ear bone. The brittleness is not a problem since the ear bone is deep within the skull. Conversely, antler is subjected to repeated severe impacts during use, so toughness is beneficial.
Yielding and Plastic Deformation
Wet bone when loaded sufficiently exhibits a yield point, sy ¼ 114 MPa (13). Beyond this critical stress level, the material does not recover upon the release of the load; permanent or plastic deformation has occurred. In bone as in most materials that exhibit yielding, the yield point is approximated by the proportional limit. The proportional limit is the boundary between the linear and nonlinear portions of the stress–strain curve. The mechanism for yield in bone differs from that in metals. In bone, yield occurs as a result of microcracking and other microdamage while in metals, yield results from motion of dislocations. Published reports have not been in agreement as to the amount of plastic deformation in bone. Much of the disagreement has been attributed to the fact that the observed
plastic deformation is highly sensitive to the hydration of the bone. Dry specimens or specimens with dried surfaces or dried and rewetted surfaces behave in a much more brittle manner than those which have been kept fully hydrated during preparation and testing. In the latter case (9), the maximum strain at fracture of bone under tension in the longitudinal direction is 0.031. Yielding of bone has considerable relevance to the function of bone in the body since very much mechanical energy can be absorbed in plastic deformation without fracture. In certain injuries, plasticity in bone can result in residual deformation, which is obvious on a clinical radiograph (53).
Bone Strain In Vivo
To ascertain the significance of bone elasticity and strength data in connection with the function of bone in the body, it is desirable to know what levels of stress and strain occur in bones during normal activities and under traumatic conditions. It is possible to make inferences from macroscopic measurements of forces acting on the extremities. The validity of such inferences is rendered uncertain by the fact that muscle forces cannot generally be uniquely determined. It has become possible to determine bone strains explicitly in various animals (54) and in humans (55) by directly cementing foil strain gages to bone surfaces (56). In a human volunteer, maximum strain along the tibia axis was 3.5 10 4 during normal walking at 1.4 m s 1 and 8 10 4 during running at 2.2 m s 1. Strains of similar magnitudes have been observed in animals such as sheep (53). The largest strain magnitude observed in the normal activity of an animal was 3.2 10 3 in the tibia of a galloping horse (57). In comparison (5), in tension in the longitudinal direction human bone yields at a strain of 6.7 10 3 and fractures at a strain of 0.03. The strain levels observed in vivo are significant in view of the fatigue properties of bone. Race horses can experience bone strain exceeding 4.8 10 3 at maximum effort (58).
Fatigue
Bone, like other materials, accumulates damage when loaded repeatedly and this damage can lead to fracture at a lower stress than the ultimate strength measured using a single load cycle. The results of in vitro mechanical fatigue studies on dead bone suggest that bone may accumulate significant fatigue damage during normal daily activity. Biological bone remodelling is concluded to be essential to the long-term structural integrity of the skeletal system (59). It is notable that dead bone, unlike steel, does not exhibit an endurance limit. The endurance limit is defined as a stress below which the material can withstand an unlimited number of cycles of repetitive load without breaking in fatigue. In the absence of an endurance limit, dead bone subjected to the prolonged cyclic load of daily activity must eventually break. Living bone, by contrast, is able to repair microdamage generated during fatigue.
The resistance of human cortical bone to fatigue fracture is more strongly controlled by strain range than stress range. Fatigue strength shows a weak positive correlation with bone density and with bone modulus and a weak negative correlation with porosity (59). Fati-
BONE AND TEETH, PROPERTIES OF |
531 |
gue strength in uniaxial tests is lower than in bending tests. In immature bone (60), bone fatigue resistance for a given strain range decreases with maturation, while the elastic modulus, density, and ash content increase with maturation. Cracking or fracture of bone due to fatigue manifests itself clinically as ‘stress fractures’ that can occur in individuals who suddenly increase their level of physical activity (61). In the case of a person in poor physical condition who sustains a fatigue fracture during military training, it is called a ‘‘march fracture’’. Laboratory results for fatigue in bone are not inconsistent with clinical experience with fatigue fractures in athletes and military recruits (62). In particular, a recruit may accumulate in 6 weeks of training a load history equivalent to 100–1000 miles of very rigorous exercise, associated with peak bone strains of 0.002 and a maximum strain range of 0.004, which can be sufficient to precipitate a fatigue fracture (62).
Stress Concentrations
It is common practice in orthopedic surgery to drill holes in bones for the placement of screws. Such holes cause the bone to be weaker than an intact bone, so that it may fracture as a result of less trauma than would ordinarily be required (63,64). This phenomenon may be understood in view of the fact that holes in elastic solids are known to cause a concentration of stress in the vicinity of the hole. According to the theory of elasticity, the stress concentration is not dependent on a decrease in the amount of material available to bear the load; it can be severe even for small holes. Laboratory studies of whole bone fracture have disclosed that a 3 mm diameter hole weakens a tibia by 40% in bending and 12% in torsion (65). A 2.8-or a 3.6- mm hole reduces the strength of dog bones (63) under rapidly applied torsion by a factor of 1.6. It is of interest to compare the observed stress concentration with predicted values based on the theory of elasticity. The predicted stress concentration factor for a hole in a field of shearing stress is 4.0 provided that the hole is small compared with the structure as a whole (66). The discrepancy has been attributed to the fact that intact bone already has stress raisers by virtue of its heterogeneous structure and porosity (63). However, in specimens loaded in the linear elastic range, well below yield or fracture, distributions and concentrations of strain differ from predicted values (67). Similar discrepancies have been reported in manmade fibrous composites without preexisting porosity (68,69). The role of the fibrous architecture of bone in relation to stress concentrations is discussed in the section on composite properties of bone.
Viscoelasticity
Bone exhibits viscoelastic behavior, that is, the stress depends not only on the strain, but also on the time history of the strain. Such behavior can manifest itself as creep, which is a gradual increase in strain under constant stress; stress relaxation, which is a gradual decrease in stress in a specimen held at constant strain; load-rate dependence of the stiffness; attenuation of sonic or ultrasonic waves; or energy dissipation in bone loaded dynamically (10).
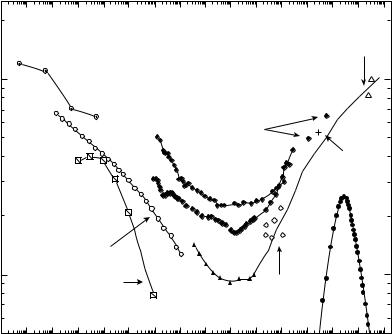
532 BONE AND TEETH, PROPERTIES OF
Experimental modalities based on each of the above phenomena have been used in the study of bone (70–74). The results have been converted to a common representation via the interrelationships inherent in the linear theory of viscoelasticity, to permit a direct comparison of results (75,76). In the case of tension–compression, there is very significant disagreement among the published results. This disagreement may result from nonlinear viscoelastic behavior not accounted for in the transformation process, or from experimental artifacts. In the case of shear deformation, however, there is good agreement between results obtained in different kinds of experiments. The loss tangent, which is proportional to the ratio of energy dissipated to energy stored in a cycle of deformation, achieves a minimum value of 0.01 at frequencies from 1 to 100 Hz. At lower and higher frequencies, the loss tangent, hence the magnitude of viscoelastic effects is greater (e.g., 0.08 at 1 MHz and at 1 mHz). To compare, the loss tangent of quartz may be < 10 6, in metals, from 10 4 to 0.01, in hard plastics from 0.01 to 0.1, and in soft polymers, it may attain values > 1. It is notable that the minimum energy dissipation in bone occurs in a frequency range characteristic of load histories during normal activities.
A synopsis of wet bone viscoelastic behavior in shear is presented in Fig. 7. The tan d attains a broad minimum over the frequency range associated with most bodily activities. Some authors have suggested that the viscoelastic behavior in bone confers a shockabsorbing role. The observed minimum in damping at frequencies of normal activities is not supportive of such an interpretation.
Some authors refer to three element spring dashpot models used in tutorials on viscoelasticity. The behavior of such a model corresponds to a Debye peak in tan d, also
shown in the figure. This corresponds, by Fourier transformation, to a single exponential in the creep or relaxation behavior. The tan d of bone occupies a much larger region of the frequency domain than a Debye peak, so the spring dashpot model is not appropriate.
Compact Bone as a Composite Material
Early composite models (83–87) for bone were two-phase models involving the mineral and protein phases only. At the ultrastructural level one may imagine the mineral crystals as a particulate reinforcing phase and the surrounding collagen as a matrix phase. Strong arguments were presented that bone cannot be described simply as a compound bar or as a material similar to prestressed concrete. Based on measured Young’s moduli of 114 GPa for hydroxyapatite (24) and 1.2 GPa for collagen derived from tendon (83), rigorous upper and lower bounds on the elastic modulus of compact bone were calculated (85,86). Young’s modulus of bone lay between these bounds and was less than the value obtained by a simple rule of mixtures approach. Similar comparisons were made for other natural collagen-apatite composites such as dentin and enamel from human teeth.
The wide disparity in the upper and lower bounds in this approach limited its applicability. Later, a composite model of the elastic properties of cortical bone was attempted in order to explain the angular dependence of the elastic properties of both wet and dried bovine cortical bone as determined in an ultrasonic wave propagation experiment (87). However, this modeling failed due to the much stronger decay in the angular dependence of the elastic modulus calculated than was found experimentally. Both this
Figure 7. Viscoelastic behavior of wet compact bone in shear. Comparison of results of various authors, adapted from (3). Low frequency tan d inferred from slope of long-term creep by Park and Lakes, 1986 (77), center circles, . Results calculated from integration of constitutive equation of Sasaki et al. 1993 (78) for torsion relaxation in bovine bone (slant squares, &). Damping adapted from data of Lakes et al., 1979 (75) for wet human tibial bone at 378C (Calculated from relaxation, circles, *; directly measured, ~). Direct tan d measurements by Garner et al., 2000 (79) for wet human bone in torsion, diamonds, !. Damping data of Thompson, 1971 (80) for whole dog radius at acoustic frequencies (diamonds, &). Damping of wet human femoral bone by Lakes, 1982 (81) via a piezoelectric ultrasonic oscillator (cross, þ). Damping at ultrasonic frequency for canine bone by Adler and Cook (1975) (82) at room temperature (open triangles, ~). Theoretical debye peak corresponding to an exponential in the time domain, solid circles .
|
Park and Lakes |
|
|
|
Adler, Cook |
|
|
Shear |
|
canine |
|
|
Human |
|
|
||
|
transformed |
|
deformation |
|
ultrasonic |
|
from creep |
|
|
|
|
0.1 |
|
|
|
|
|
|
|
|
resonant |
|
Lakes |
|
|
|
|
|
|
δ |
|
|
Garner et al. |
|
human |
|
|
|
piezoelectric |
||
tan |
|
|
human |
|
ultrasonic |
|
|
|
transverse |
|
|
|
Lakes et al. |
|
|
|
|
|
human |
|
|
|
|
|
transformed |
|
|
|
|
|
from relaxation |
|
Longitudinal |
|
|
|
|
|
|
|
|
0.01 |
Sasaki et al., |
|
Thompson, |
|
|
|
|
|
|||
|
bovine, transformed |
|
|||
|
Lakes et al. |
whole |
|
||
|
from relaxation |
|
Debye |
||
|
|
human |
canine |
||
|
|
|
|||
10−8 10−7 10−6 10−5 10−4 |
10−3 10−2 10−1 100 101 102 |
103 104 |
105 106 107 |
||
|
|
|
Frequency (Hz) |
|
|
attempt (88) and the earlier calculations (84–87) failed to model the properties of compact bone because they did not incorporate the dependence of its properties on its complex hierarchical structure. Inclusion of the hierarchical structural organization of bone was accomplished by adaptation of the hollow fiber composite model (89) so that it resembled the structure of Haversian bone with the osteons viewed as hollow fibers embedded in a matrix (36,37). Subsequently, the same model (35,36,89) was adapted to calculate the viscoelastic properties of bone (90). In recent years, homogenization techniques have been applied to obtain even further improvements In the composite modeling (91,92).
The matrix is the ground substance that comprises the cement lines between osteons and is thought to be principally composed of mucopolysaccharides. The stiffness of the ground substance has not been determined experimentally, but it has been inferred from the composite model in conjunction with experimental data from the ultrasound studies on whole bone (93,94). Based on this calculation, the ground substance was computed to be about one-quar- ter as stiff as the osteon itself. The view of the ground substance as a compliant interface is also supported by the results of several experimental studies. Localized slippage occurs at the cement lines in specimens of bovine plexiform bone (95) of human Haversian bone (77) subjected to prolonged stress. Under such conditions, the ground substance at the cement lines appears to behave in a viscous manner. Considering the full range of load rates and frequencies, one may view the ground substance as a compliant and highly viscoelastic material. Such a conclusion is perhaps surprising (2) in view of the fact that the ground substance is highly mineralized (96). Nevertheless a view of the ground substance as a compliant interface is supported by further experiments. For example, studies of single osteons and osteon groups in torsion have revealed size effects to occur and the osteon to have a higher effective shear modulus than whole bone (97). Similar size effects were observed in torsional and bending experiments upon larger microsamples (98,99). These latter results have been interpreted in light of a generalized form of elasticity theory, known as Cosserat elasticity, which admits both strain of the material and local rotations of microscopic constituents, for example, the osteons (97–99). A twist per unit area or couple stress can occur in addition to a force per unit area or couple stress. By contrast, classical elasticity (Eq. 1), which describes most ordinary materials, involves only strains and stresses. Cosserat elasticity is likely differ significantly from classical elasticity in its predictions of stress and strain around holes, cracks, and interfaces. For large, whole bones, conventional anisotropic elasticity has been shown to be entirely adequate (39).
The interface between osteons also appears to be important in conferring a measure of fracture toughness upon compact bone. In particular, the crack blunting mechanism of Cook and Gordon (100) confers toughness in fibrous media by the action of a weak interface between fibers in blunting a propagating crack (101). Evidence for the role of the cement substance as such a weak interface has been presented by Piekarski (102). Pullout of fibers can result in a large energy absorption in the fracture of fibrous compo-
BONE AND TEETH, PROPERTIES OF |
533 |
sites (103). This toughening mechanism also appears to be operative in compact bone, as seen in micrographs of pullout of osteons in fractured bone specimens (51,104).
Consideration of bone as a composite material may provide insight regarding various phenomena in the mechanics of bone. In particular, stress concentration around holes is significantly less than the predicted value (63). The strength of notched specimens does not decrease with the sharpness of the notch as expected; instead, the strength is independent of notch sharpness (49). Residual strain around holes in bone does not follow the predictions of classical anisotropic elasticity (98,99). The fatigue life of bone specimens in bending exceeds that of specimens in tension by a factor of several thousand (59). Similar effects have been observed in various manmade fibrous composites such as boron-epoxy and graphite-epoxy (104). Such phenomena are not correctly predicted by elasticity theory, but may be accounted for in the context of structural models, composite theories, or generalized continuum models (77).
Polycrystalline elastic properties and single-crystal elastic constants of apatites that are useful In the modeling of calcified tissues elasticity are given in Table 6.
Mechanical Properties of Cancellous Bone
Cancellous or trabecular bone is a highly porous or cellular form of bone. In a typical long bone, the cortex or exterior of the shaft (diaphysis) and flared ends (metaphysis) is composed of compact bone while the interior, particularly near the articulating ends, is filled with cancellous bone. Cancellous bone may also be found to fill the interior of short bones and flat bones as well as in the interior of bony tuberosities under mucle attachments. The structure of cancellous bone is that of a latticework of bars and plates; typical structure is shown in Fig. 3. The volume fraction of solid material can be from 5 to 70%; the interstices are filled with marrow. The compressive strength sult(in MPa) depends very much on the density r (in g cm 3) and also varies with the strain rate de/dt (in s 1) as follows (105).
sult ¼ 68ðde=dtÞ0:06r2
This relation also models the compressive strength of compact bone (221 MPa, at a density of r. ¼ 1.8 g cm 3). To a certain degree of approximation, both compact and cancellous bone may be mechanically viewed as a single material of variable density (105). Density is not, however, the only determinant of the properties of cancellous bone. The microstructure can vary considerably from one part of the body to another (106). For example, in the vertebras and in the tibia (107), a highly oriented, columnar architecture is observed. This kind of trabecular bone is highly anisotropic: the Young’s modulus in the longitudinal direction can exceed that in the transverse direction by more than a factor of 10 (107). By contrast, in regions such as the proximal part of the bovine humerus, the cancellous bone can be essentially isotropic (108). This bone is about twice as strong in compression as in tension. In many ways, cancellous bone (109– 111) is similar in its behavior to manmade rigid cellular foams (112). For example, in compression, the stress–strain curves contain a linear elastic region, up to a strain of 0.05, at which the cell walls bend or compress (112). A plateau
534 BONE AND TEETH, PROPERTIES OF
region of almost constant macroscopic stress is associated with elastic buckling, plastic yield, or fracture of the cell walls. The compressive failure of the cancellous bone proceeds at approximately constant stress until the cell walls touch each other; at this point any further compression causes the stress to rise rapidly (112). By contrast, fracture of cancellous bone in tension proceeds abruptly and catastrophically (108). The energy absorption capacity of cancellous bone is consequently much less in tension than it is in compression (109). This suggests that tensile and avulsion fractures of cancellous bone observed clinically are associated with minimal energy absorption, and therefore may be precipitated by relatively minor trauma (109). The elastic modulus E for cancellous bone increases as the square of the density (E ¼ kr2) if the structure consists of open cells forming a network of rods (110,111). In closed-cell cancellous structures consisting of plates, the modulus E is proportional to the cube of the density (E ¼ kr3). Based on study of micrographs and density maps of femora and vertebras, it is suggested that an open cell structure of rods is found when the solid volume fraction is less than 0.13, while a closed cell plate structure occurs at a density of > 350 kg m 3 corresponding to a relative density or solid volume fraction of 0.20 (110). The relationship between density and structure may not be so straightforward in all situations. As for the highly oriented columnar cancellous bone from the human tibia (107), the modulus E in the longitudinal direction is proportional to the density (E ¼ kr). Such behavior is anticipated on the basis of an axial compressional mode of deformation of the cell walls, in contrast to the bending mode that is expected in rod and plate structures (112).
It is important to distinguish the difference between the elastic moduli of volumetric samples of trabecular bone that are highly porous, low density structures, thus exhibiting low moduli, generally well below 1.0 GPa as obtained by mecanical testing techniques, and that of individual trabeculae, comparable in structure and density to cortical bone,thus exhibiting much higher moduli (10–20 GPa), as obtained in recent years by nanoindentation (19) and SAM (18) in addition to that obtained by mechanical testing). Values of the elastic moduli of both trabecular volumes and individual trabeculae are given in Table 7.
ADAPTIVE PROPERTIES OF BONE
Phenomenology
The relationship between the mass and form of a bone to the forces applied to it was appreciated by Galileo (113), who is credited with being the first to understand the balance of forces in beam bending and with applying this understanding to the mechanical analysis of bone. Wolff (114) published his seminal 1892 monograph on bone remodeling; the observation that bone is reshaped in response to the forces acting on it is presently referred to as Wolff’s law. Cowin, in Chapter 25 of Ref. 3, discussed ‘‘The Problems with Wolff’s Law ’’. Many relevant observations regarding the phenomenology of bone remodeling have been compiled and analyzed by Frost (115,116). Salient points are as follows:
1. Remodeling is triggered not by principal stress but by ‘‘flexure’’.
2. Repetitive dynamic loads on bone trigger remodelling; static loads do not.
3. Dynamic flexure causes all affected bone surfaces to drift toward the concavity that arises during the act of dynamic flexure.
These rules are essentially qualitative and they do not deal with underlying causes. A critique of these ideas has been presented by Currey (1,2). Additional aspects of bone remodeling may be found in the clinical literature. For example, after complete removal of a metacarpal and its replacement with graft consisting of a strut of tibial bone, the graft becomes remodeled to resemble a real metacarpal; the graft continues to function after 52 years (117). In the standards of the Swiss Association for Internal Fixation it is pointed out that severe osteoporosis can result from the use of two bone plates in the same region as a result of the greatly reduced stress in the bone (118). Pauwels (119) suggested that as a result of bending stresses the medial and lateral aspects of the femur should be stiffer and stronger than the anterior and posterior aspects. Such a difference has actually been observed (120). Large cyclic stress causes more resorption than large static stress (121). Immobilization of humans causes loss of bone and excretion of calcium and phosphorus (122). Long spaceflights under zero gravity also cause loss of bone (123,124); hypergravity induced by centrifugation strengthens the bones of rats (125,126). Studies of stress-induced remodeling of living bone have been performed in vitro (127). Recently, in vivo studies in pigs (128) were conducted. In this study, strains were directly measured by strain gages before and after remodeling. Remodeling was induced by removing part of the pigs’ ulna so that the radius bore all the load. Initially, the peak strain in the ulna approximately doubled. New bone was added until, after 3 months, the peak strain was about the same as on the normal leg bones. In vivo experiments conducted in sheep (129) have disclosed similar results. It is of interest to compare the response time noted in the above experiments with the rate of bone turnover in healthy humans. The life expectancy of an individual osteon in a normal 45 year old man is 15 years and it will have taken 100 days to produce it
(130,131).
Remodeling of Haversian bone seems to influence the quantity of bone but not its quality, that is, young’s modulus, tensile strength, and composition (132). However, the initial remodeling of primary bone to produce Haversian bone results in a reduction in strength (1,2). As for the influence of the rate of loading on bone remodeling, there is good evidence to suggest that intermittent deformation can produce a marked adaptive response in bone, whereas static deformation has little effect (127). Experiments (133) upon rabbit tibiae bear this out. In the dental field, by contrast, it is accepted that static forces of long duration move teeth in the jawbone. In this connection (134), the direction (as well as the type) of stresses acting on the bone tissue should also be considered. Currey (1,2) points out that the response of different bones in the same skeleton to mechanical loads must differ, otherwise lightly loaded
bones such as the top of the human skull, or the auditory ossicles, would be resorbed.
Failure of bone remodeling to occur normally in certain disease states is of interest: for example, osteropetrotic bone contains few if any viable osteocytes and usually contains a much larger number of microscopic cracks than adjacent living bone (135). This suggests that the osteocytes play a role in detecting and repairing the damage. In senile osteoporosis, bone tissue is removed by the body, often to such an extent that fractures occur during normal activities. Osteoporosis may be referred to as a remodeling error (116).
Some theoretical work, notably by Cowin and others (3,136) has dealt with the problem of formulating Wolff’s law in a quantitative fashion. In this theory, constitutive equations are developed, which predict the remodeling response to a given stress. Stability considerations are invoked to obtain some constraints on the parameters in the constitutive equation.
Feedback Mechanisms
Bone remodeling appears to be governed by a feedback system in which the bone cells sense the state of strain in the bone matrix around them and either add or remove bone as needed to maintain the strain within normal limits. The process or processes by which the cells are able to sense the strain and the important aspects of the strain field are presently unknown. Bassett and Becker (137) reported that bone is piezoelectric, that is, that it generates electric fields in response to mechanical stress; they advanced the hypothesis that the piezoelectric effect is the part of the feedback loop by which the cells sense the strain field. This hypothesis obtained support from observations of osteogenesis in response to externally applied electric fields of the same order of magnitude as those generated naturally by stress via the piezoelectric effect. The study of bone bioelectricity has received impetus from observations that externally applied electric or electromagnetic fields stimulate bone growth (138). The electrical hypothesis, while favored by many, has not been proven. Indeed, other investigators have advanced competing hypotheses that involve other mechanisms by which the cells are informed of the state of stress around them.
For example, inhomogeneous deformation at the lamellae may impinge on osteocyte processes and thus trigger the osteocytes to initiate bone formation or remodelling (139). Motion at the cement lines was observed and it was suggested that such motion could act as a passive mechanism by which bone’s symmetry axes may become aligned to the direction of time averaged principal stresses (99). Stress on bone may induce flow of fluid in channels, (e.g., canaliculi), and such flow could play a role in the nutrition and waste elimination of osteocytes, which may be significant in bone remodeling (140). In a related vein, theoretical arguments have been presented in support of the hypothesis that bone cells are directly sensitive to hydrostatic pressure transmitted to them from the bone matrix via the tissue fluid (141). Although no experimental test of this direct pressure hypothesis has been published, we observe with interest that direct hydrostatic pressure
BONE AND TEETH, PROPERTIES OF |
535 |
has been observed to alter the swimming behavior of paramecia, possibly by means of action upon the cell membrane (142). Otter and Salman found that a hydrostatic pressure of 68 atm abolishes the reversing of direction of swimming, 170 atm stops swimming, and 400–500 atm irreversibly damages the cell. We observe that 100 atm corresponds to 1400 psi stress, or in bone, a strain of 0.07%, which is in the normal range of bone strain and 500 atm corresponds to 7000 psi or a strain of 0.35%, well above the normal range of bone strain. Stress in bone also results in temperature differences between osteons (143); the cells may be sensitive to sudden temperature changes during human activity. A mechanochemical hypothesis has been advanced, in which the solubility of calcium may be affected by stress in the bone matrix (144). Strain energy in bone might also influence the energetics of bone mineral nucleation (145). It has also been suggested that remodeling may be initiated in response to microcracks generated by mechanical fatigue of bone (146). In summary, many hypotheses have been proposed for the mechanism by which appropriate cells sense the state of strain in bone, but little or no experimental evidence is available to discriminate among them.
Cellular and Biochemical Aspects of Bone Remodeling
The adaptive response of bone to mechanical stimuli is mediated by living cells. A great deal is known concerning bone cell function and its control by ionic and hormonal factors, but little is known concerning the effect of mechanical strain in bone upon the biochemistry of its cells. Rasmussen and Bordier (147) have presented an extensive review of studies of bone cell physiology. Recently, the biochemical consequences of electrical stimulation of bone have been reported (148). Biochemical steps associated with cell activation are as yet poorly understood, but ion fluxes appear to play a role (149). Cyclic nucleotides mediate the effects of extracellular signals (150) and prostaglandins modulate them (149). Prostaglandin E2 has been hypothesized to mediate bone resorption in trauma, malignancy, and periodontal disease. This prostaglandin, as well as the cellular constituents cyclic AMP and cyclic GMP, has been found in association with regions of bone stimulated electrically (148).
ELECTRICAL PROPERTIES OF BONE
Fukada and Yasuda (151) first demonstrated that dry bone is piezoelectric in the classic sense, that is, mechanical stress results in electric polarization, the indirect effect; and an appplied electric field causes strain, the converse effect. The piezoelectric properties of bone are of interest in view of their hypothesized role in bone remodeling (137). Wet collagen, however, does not exhibit piezoelectric response. Studies of the dielectric and piezoelectric properties of fully hydrated bone raise some doubt as to whether wet bone is piezoelectric at all at physiological frequencies (152). Piezoelectric effects occur in the kilohertz range, well above the range of physiologically significant frequencies (152). Both the dielectric properties (153) and the piezoelectric properties of bone (154) depend strongly on
536 BONE AND TEETH, PROPERTIES OF
frequency. The magnitude of the piezoelectric sensitivity coefficients of bone depends on frequency, on direction of load, and on relative humidity. Values up to 0.7 pC/N have been observed (154), to be compared with 0.7 and 2.3 pC/N for different directions in quartz, and 600 pC/N in some piezoelectric ceramics. It is, however, uncertain whether bone is piezoelectric in the classic sense at the relatively low frequencies which dominate in the normal loading of bone. The streaming potentials examined originally by Anderson and Eriksson (155,156) can result in stress generated potentials at relatively low frequencies even in the presence of dielectric relaxation, but this process is as yet poorly understood.
Potentials observed in bent bone differ from predictions based on the results of experiments performed in compression (157). The piezolectric polarization may consequently depend on the strain gradient (157) as well as on the strain. This piezoelectric theory has been criticized as ad hoc by some authors, however, the idea has some appeal in view of Frost’s modeling (115,116) and Currey’s suggestion (1,2) that strain gradients may be significant in this regard. The gradient theory is not ad hoc, but can be obtained theoretically from general nonlocality considerations (158). The physical mechanism for such effects is hypothesized to lie in the fibrous architecture of bone (26,78). Theoretical analyses of bone piezoelectricity (159–162) may be relevant to the issue of bone remodeling. Recent thorough studies have explored electromechanical effects in wet and dry bone. They suggest that two different mechanisms are responsible for these effects: Classical piezoelectricity due to the molecular asymmetry of collagen in dry bone, and fluid flow effects, possibly streaming potentials in wet bone (163).
Bone exhibits additional electrical properties which are of interest. For example, the dielectric behavior (e.g., the dynamic complex permittivity) governs the relationship between the applied electric field and the resulting electric polarization and current. Dielectric permittivity of bone has been found to increase dramatically with increasing humidity and decreasing frequency (152,153). For bone under partial hydration conditions, the dielectric permittivity (which determines the capacitance) can exceed 1000 and the dielectric loss tangent (which determines the ratio of conductivity to capacitance) can exceed unity. Both the permittivity and the loss are greater if the electric field is aligned parallel to the bone axis. Bone under conditions of full hydration in saline behaves differently: the behavior of bovine femoral bone is essentially resistive, with very little relaxation (164). The resistivity is 45–48 mm for the longitudinal direction, and three to four times greater in the radial direction. These values are to be compared with a resistivity of 0.72 mm for physiological saline alone. Since the resistivity of fully hydrated bone is 100 times greater than that of bone under 98% relative humidity, it is suggested that at 98% humidity the larger pores are not fully filled with fluid (164).
Compact bone also exhibits a permanent electric polarization as well as pyroelectricity, which is a change of polarization with temperature (165,166). These phenomena are attributed to the polar structure of the collagen molecule; these molecules are oriented in bone. The orientation of
permanent polarization has been mapped in various bones and has been correlated with developmental events.
Electrical properties of bone are relevant not only as a hypothesized feedback mechanism for bone remodeling, but also in the context of external electrical stimulation of bone to aid its healing and repair (167,168).
The frequency of electrical stimulation influences its effectiveness (169). A frequency band of 20–30 Hz was found from analysis of strain data. Bone growth could be stimulated more easily in the avian ulna at relatively higher frequencies. Electromagnetic stimuli also prevent bone loss due to disuse as revealed in an isolated canine fibula model (170). Bone density may be maintained and increased by weight lifting, which involves no medical intervention. Indeed, bone mineral content values (as determined with dual photon absorptiometry) in the spines of athletes were extremely high and were closely correlated to the amount of weight lifted during training (171).
BIBLIOGRAPHY
Cited References
1.Currey J. The mechanical adaptations of bones. Princeton: Princeton University Press; 1984.
2.Currey J. Bone Structure and Mechanics. Princeton: Princeton University Press; 2002.
3.Cowin S. Bone Mechanics. 2nd ed. Boca Raton, FL: CRC Press; 2001.
4.Park JB, Lakes RS. Biomaterials. 2nd ed. New York: Plenum; 1992.
5.Hancox NM. Biology of Bone. Cambridge, (MA): Cambridge University Press; 1972.
6.LeGeros RZ. Monographs in Oral Science. Karger: 1991.
7.Sokolnikoff IS. Mathematical theory of elasticity. Krieger; 1983.
8.Ferracane JL. Materials In Dentistry. 2nd ed. Philadelphia: Lippincott, Williams & Wilkins; 2001.
9.Briggs A. Acoustic Microscopy. Oxford: Clarendon Press; 1992.
10.Lakes RS. Viscoelastic Solids. Boca Raton, FL: CRC Press; 1998.
11.Eppell SJ, Tong WL, Katz JL, Kuhn L, Glimcher MJ. Shape and size of isolated bone mineralites measured using atomic force microscopy’’ J Ortho Res 2001;19:1027–1034.
12.Tong W, Glimcher MJ, Katz JL, Kuhn L, Eppell SJ. Size and shape of Mineralites in young bovine bone measured by atomic force microscopy. Calcif Tiss Inter 2003;72:592–598.
13.Reilly DT, Burstein AH. The elastic and ultimate properties of compact bone tissue. J Biomech 1975;8:393–405.
14.Craig RG, Peyton FA. Elastic and mechanical properties of human dentin. J Dental Res 1958;37:710–718.
15.Craig RG, Peyton FA. Compressive properties of enamel, dental cements, and gold. J Dental Res 1961;40:936–945.
16.Ashman RB, Cowin SC, Van Buskirk WC, Rice JC. A continuous wave technique for the measurement of the elastic properties of cortical bone. J Biomech 17:349–361.
17.Reilly DT, Burstein AH. The mechanical properties of cortical bone. J Bone Jnt Surg 1974;56A:1001–1022.
18.Bumrerraj S, Katz JL. Scanning acoustic microscopy study of human cortical and trabecular bone. Ann Biomed Eng 2001;29:1–9.
19.Rho JY, Pharr GM. Effects of drying on the mechanical poroperties of bovine femur measured by nanoindentation. J Mater Sci, Matyer Med 1999;10:1–4.
20.Kapur R. The use of scanning acoustic microscopy to study the microstructural properties of the dentin/enamel junction, B.S. Project (Katz JL, Advisor), Department of Biomedical Engineering, Case Western Reserve University; 1999.
21.Lo¨st C, Irion KM, Nussle JC. Two-dimensional distribution of sound velocity in ground sections of enamel. Endod Dent Traumatol 1992;8:215–218.
22.Van Buskirk WC, Ashman RB. The elastic moduli of bone. In: Mechanical Properties of Bone, Joint ASME-ASCE Applied Mechanics, Fluids Engineering and Bioengineering Conference. Boulder, CO; 1981.
23.Lees S, Rollins FR, Jr. Anisotropy in hard dental tissues. J Biomech 1972;5:557–566.
24.Lees S, Ahern JM, Leonard M. Parameters influencing the sonic velocity in compact calcified tissues of various species. J Acoust Soc Am 1983;74:28–33.
25.Gilmore RS, Pollack RP, Katz JL. The elastic properties of
bovine dentin and enamel. Arch Oral Biol 1970;15:787– 796.
26.Katz JL, Lipson S, Yoon HS, Maharidge R, Meunier A, Christel P. The effects of remodeling on the elastic properties of bone, Calc Tiss Inter 1984;36:S31–S36.
27.Yoon HS, Katz JL. Ultrasonic wave propagation in human cortical bone. II. Measurements of elastic properties and microhardness. J Biomech 1976;9:459–464.
28.Yoon HS, Newnham RE. Elastic Properties of fluorapatite. Am Min 1969;54:1193–1197.
29.Gordon JE. Structures. Penguin; 1983.
30.Lemons RA, Quate CF. Acoustic microscopy-scanning version. Appl Phys Lett 1974;24:163–165.
31.Lemons RA, Quate CF. Acoustic microscopy. Phys Acoust 1979;14:1–92.
32.Katz JL, Meunier A. Scanning acoustic microscope studies of the elastic properties of osteons and osteon lamellae. J Biomech Eng 1993;115:543–548.
33.Evans FG, Bang S. Differences and relationships between the physical properties and the microscopic structure of human femoral, tibial, and fibular bone. Am J Anat 1967;120:79–88.
34.Evans FG, Vincentelli R. Relations of the compressive properties of human cortical bone to histological structure and calcification. J Biomech 1974;7:1–10.
35.Lang SB. Ultrasonic method for measuring elastic coefficients of boneand results on fresh and dried bovine bones. IEEE Trans Biomed Eng, BME 1970;17:101–105.
36.Yoon HS, Katz JL. Ultrasonic wave propagation in human cortical bone, I. Theoretical considerations for hexagonal symmetry, J Biomech 1976;9:407–412.
37.Katz JL. Hierarchical modeling of compact Haversian bone as a fiber reinforced material. In: 1976 Advances in Bioengineering. New York City: ASME; 1976. p 17–18.
38.Katz JL. On the anisotropy of young’s modulus of bone. Nature (London) 1980;283:106–107.
39.Huiskes R, Janssen JD, Sloof TJ. A detailed comparison of experimental and theoretical stress analyses of a human femur. In: Mechanical Properties of Bone, Joint ASME-ASCE Applied Mechanics, Fluids Engineering and Bioengineering Conference. Boulder, (CO); 1981.
40.Lipson SF, Katz JL. The relationship between the elastic properties and microstructure of bovine cortical bone. J Biomech 1984;17:231–240.
41.Ashman RB, Rosina G, Cowin SC, Fontenot MG. The bone tissue of the canine mandible is elastically isotropic. J Biomech 1985;18:717–721.
42.Ashman RB, Van Buskirk WC, Cowin SC, Sandbornj PM, Wells MK, Rice JC. The mechanical Properties of immature osteopetrotic bone. Calc Tiss Inter 1985;37:73–76.
43.Lakes RS. Materials with structural hierarchy. Nature (London) 1993;361:511–515.
BONE AND TEETH, PROPERTIES OF |
537 |
44.Melick RA, Miller DR. Variations of tensile strength of human cortical bone with age. Clin Sci 1966;30:243–248.
45.Hazama H. Study of the torsional strength of the compact substance of human beings. J Kyoto Pref Med Univ 1956;60:167–184.
46.Evans FG, Lebow M. Regional differences in some of the physical properties of the human femur. J Appl Physiol 1951;3:563–572.
47.Sedlin ED, Hirsch C. Factors affecting the determination of the physical properties of femoral cortical bone. Acta Orthop Scand 1966;37:29–48.
48.Burstein AH, Reilly DT, Martens M. Aging of bone tissue: mechanical properties. J Bone Jnt Surg 1976;58A:82–86.
49.Bonfield W, Datta PK. Fracture toughness of compact bone. J Biomech 1976;9:131–134.
50.Behiri JC, Bonfield W. Crack velocity dependence of longitudinal fracture in bone. J Mat Sci 1980;15:1841–1849.
51.Currey JD. Changes in the impact energy absorption of bone with age. J Biomech 1979;12:459–469.
52.Corondan G, Haworth WL. A fractographic study of human long bone. J Biomech 1986;19:207–218.
53.Carter DR, Spengler DM. Biomechanics of fracture. In: Sumner Smith G, editor. Bone in Cinical Orthopaedics. New York: Saunders; 1982. p 305–332.
54.Lanyon LE. Analysis of surface bone strain in the calcaneus of sheep during normal locomotion. J Biomech 1973;6:41– 69.
55.Lanyon LE, Hampson WGJ, Goodship AE, Shah JS. Bone deformation recorded in vivo from strain gauges attached to the human tibial shaft. Acta Orthop Scand 1975;46:256–268.
56.Caler WE, Carter DR, Harris WH. Techniques for implementing an in vivo bone strain gage system. J Biomech 1981;14:503–507.
57.Rubin CT. Skeletal strain and the functional significance of bone architecture. Calcif Tissue Int 1984;36:S11–S18.
58.Nunamaker DM, Butterweck DM, Provost MT. Fatigue fractures in thoroughbred racehorses: relationships with age, peak bone strain, and training. J Orthop Res 1990;8:694.
59.Carter DR, Caler WE, Spengler DM, Frankel VH. Uniaxial fatigue of human cortical bone. The influence of tissue physical characteristics. J Biomech 1981;14:461–470.
60.Keller TS, Lovin JD, Spengler DM, Carter DR. Fatigue of immature baboon cortical bone. J Biomech 1985;18:297–304.
61.Devas MB. Stress fractures. Churchill Livingstone, London; 1975.
62.Carter DR, Caler WE, Spengler DM, Frankel VH. Fatigue behavior of adult cortical bone: the influence of mean strain and strain range. Acta Orthop Scand 1981;52:481–490.
63.Brooks DB, Burstein AH, Frankel VH. The biomechanics of torsional fractures: the stress concentration effect of a drill hole. J Bone Jnt Surg 1970;52A:507–514.
64.Burstein AH, Currey JD, Frankel VH, Heiple KG, Lunseth P, Vessely JC. Bone strength: the effect of screw holes. J Bone Jnt Surg 1972;54A:1143–1156.
65.Laurence M, Freeman MA, Swanson SA. Engineering considerations in the internal fixation of fractures of the tibial shaft. J Bone Jnt Surg 1969;51B:754–768.
66.Timoshenko S, Goodier JM. Theory of elasticity, 3rd ed. New York: McGraw Hill; 1983.
67.Lakes RS, Yang JFC. Concentration of strain around holes in a strip of compact bone, Developments in mechanics, Proceedings of the 18th Midwestern Mechanics Conference. Volume 12, Iowa City; 1983. p 233–237.
68.Awerbuch J, Madhukar S. Notched strength of composite laminates: predictions and experiments, a review. J Reinforced Plast Comp 1985;4:3–159.
69.Daniel IM. Strain and failure analysis in graphite/epoxy plates with cracks. Exper Mech 1978;18:246–252.
538BONE AND TEETH, PROPERTIES OF
70.Smith R, Keiper D. Dynamic measurement of viscoelastic properties of bone. Am J Med Electr 1965;4:156.
71.Currey JD. Anelasticity in bone and echinoderm skeletons. J Exper Biol 1965;43:279.
72.Black J, Korostoff E. Dynamic mechanical properties of viable human cortical bone. J Biomech 1973;16:435.
73.Tennyson RC, Ewert R, Niranjan V. Dynamic viscoelastic response of bone. Exp Mech 1972;12:502.
74.Lugassy AA, Korostoff E. Viscoelastic behavior of bovine femoral cortical bone and sperm whale dentin. In: Research in Dental and Medical Materials. New York: Plenum; 1969.
75.Lakes RS, Katz JL, Sternstein SS. Viscoelastic properties of cortical bone: Part 1: Torsional and biaxial studies. J Biomech 1979;12:657.
76.Lakes RS, Katz JL. Interrelationships among the viscoelastic functions for anisotropic solids: application to calcified tissues and related systems. J Biomech 1974;17:259.
77.Park HC, Lakes RS. Cosserat micromechanics of human bone: strain redistribution by a hydration-sensitive constituent. J Biomech 1986;19:385–397.
78.Sasaki N, Nakayama Y, Yoshikawa M, Enyo A. Stress relaxation function of bone and bone collagen. J Biomech 1993;26:1369–1376.
79.Garner E, Lakes RS, Lee T, Swan C, Brand R. Viscoelastic dissipation in compact bone: implications for stress-induced fluid flow in bone. J Biomech Eng 2000;122:166–172.
80.Thompson G, Experimental studies of lateral and torsional vibration of intact dog radii, [dissertation]. Stanford (CA): Stanford University; 1971.
81.Lakes RS. Dynamical study of couple stress effects in human compact bone. J Biomech Eng 1982;104:6–11.
82.Adler L, Cook CV. Ultrasonic parameters of freshly frozen dog tibia. J Acoust Soc Am 1975;58:1107–1108.
83.Currey JD. Three analogies to explain the mechanical properties of bone. Biorheology 1964;2:1–10.
84.Welch DO. The composite structure of bone and its response to mechanical stress. Recent Adv Eng Sci 1970;5:245– 262.
85.Katz JL. Hard tissue as a composite material-I. Bounds on the elastic behavior. J Biomech 1971;4:455–473.
86.Piekarski K. Analysis of bone as a composite material. Inter J Eng Sci 1973;11:557–565.
87.Currey JD. The relationship between the stiffness and the mineral content of bone. J Biomech 1969;2:477.
88.Bonfield W, Grynpas MD. Anisotropy of Young’s modulus of bone. Nature (London) 1977;270:453–454.
89.Hashin Z, Rosen BW. The elastic moduli of fiber reinforced materials. J Appl Mech 1964;31:223–2xx.
90.Gottesman T, Hashin Z. Analysis of viscoelastic behavior of bone on the basis of microstructure. J Biomech 1979;13:89–yy.
91.Hogan H. Micromechanics modeling of haversian cortical bone properties. J Biomech 1992;25:549–zzz.
92.Crolet JM, Aoubiza B, Meunier A. Compact bone: Numerical simulation of mechanical characteristics. J Biomech 1993;26:677–aaa.
93.Katz JL, Maharidge RL, Yoon HS. The estimation of interosteonal mechanical properties from a composite model for haversian bone. In: Perren SM, Scheider E, editors. Biomechanics: Current Interdisciplinary Research. Dordrecht: Martinus Injhoff, 1985. p 179–184.
94.Katz JL, Maharidge RL, Yoon HS. Calculation of inter-osteo- nal mechanical properties for haversian bone based on a hierarchical composite model. Biomechanics Symp. AMDVol. 68, FED-Vol. 21. New York City: ASME; 1985. p 33–35.
95.Lakes RS, Saha S. Cement line motion in bone. Science 1979;204:501–503.
96.Frasca P. Scanning-electron microscopy studies of ‘ground substance’ in the cement lines, resting lines, hypercalcified rings, and reversal lines of human cortical bone, Acta Anatomica 1981;109:115–121.
97.Frasca P, Harper R, Katz JL. Strain and frequency dependence of shear storage modulus for human single osteons and cortical bone microsamples-size and hydration effects. J Biomech 1981;14:679–690.
98.Yang JFC, Lakes RS. Transient study of couple stress effects in human compact bone: Torsion. J Biomech Eng 1981;103:275–279.
99.Yang JFC, Lakes RS. Experimental study of micropolar and couple stress elasticity in compact bone in bending. J Biomech 1982;15:91–98.
100.Cook J, Gordon JE. A mechanism for the control of crack propagation in all-brittle systems. Proc R Soc London 1964;A282:508–520.
101.Kelly A. The strengthening of metals by dispersed particles. Proc R Soc London 1964;A282:63–79.
102.Piekarski K. Fracture of Bone. J Appl Phys 1970;41:215– 223.
103.Kelly A. Strong solids. London: Oxford University Press; 1966.
104.Wright TM, Barnett DM, Hayes WC. Residual stresses in bone. Volume 3, Recent Advances in Engineering Science.
Boston: Scientific Publishers; 1977. p 25–32, Proceedings, 10th meeting, Society of Engineering Science, NC: Raleigh; 1973.
105.Carter DR, Hayes WC. Bone compressive strength: the influence of density and strain rate. Science 1976;194: 1174–1176.
106.Dyson ED, Jackson CK, Whitehouse WJ. Scanning electron microscope studies of human trabecular bone. Nature (London) 1970;225:957–959.
107.Williams JL, Lewis JL. Properties and an anisotropic model of cancellous bone from the proximal tibial epiphysis. J Biomech Eng 1982;104:50–56.
108.Kaplan S, Hayes WC, Stone JL, Beaupre GS. Tensile strength of bovine trabecular bone. J Biomech 1985;18: 723–727.
109.Carter DR, Schwab GH, Spengler DM. Tensile fracture of cancellous bone. Acta Orthop, Scand 1980;51:733–741.
110.Gibson LJ. The mechanical behaviour of cancellous bone. J Biomech 1985;18:317–328.
111.Carter DR, Hayes WC. The compressive behaviour of bone as a two-phase porous structure. J Bone Jnt Surg 1977;59A:954–962.
112.Gibson LJ, Ashby MF. The mechanics of three-dimensional cellular materials. Proc R Soc London 1982;A382:25–42.
113.Galilei G, Discorsi E. Dimostrazioni Matematiche intorna a due nuove Scienze. 1638, Translated by H Crew, A deSalvio, editors. New York: Macmillan; pp 158–172. 1914. p 118– 134.
114.Wolff J. Das Gesetz der Transformation der Knochen. Berlin: Hirschwald; 1892.
115.Frost HM. Bone remodelling and its relation to metabolic bone diseases. Springfield, IL: C Thomas; 1973.
116.Frost HM. Bone modelling and skeletal modelling errors. Springfield, IL: C Thomas; 1973.
117.Nathan PA, Fowler A. Remodeling of a metacarpal bone graft in a child. J Bone Jnt Surg 1976;58A:719–722.
118.Muller ME, Allgouer M, Willeneger H. Manual of Internal Fixation—Technique Recommended by the AO Group. Springer Verlag; 1970.
119.Pauwels F. Die Bedeutung des Bauprinzipien des Stu¨ tz, und Bewegensapparatens fu¨ r, die Beanspruchung der Rohrenknochen. Z Anat Entwicklungs 1948;114:129–166.
120.Amtmann E. The distribution of the breaking strength in the femur. J Biomech 1968;1:271–277.
121.Seirig A, Kempko W. Behavior of in vivo bone under cyclic loading. J Biomech 1969;2:455–461.
122.Dietrick JE, Whedon G, Shorr E. Effects of immobilization upon various metabolic and physiological functions of bone. Am Jnl Med 1948;4:3–36.
123.Mack PB, La Chance PL. Effects of recumbency and space flight on bone density. Am J Clin Nutrition 1967;20:194– 205.
124.Morey ER, Baylink DK. Inhibition of bone formation during space flight. Science 1978;201:1138–1141.
125.Wunder CC, Briney SR, Skangstad CA. Growth of mouse femurs during chronic centrifugation. Nature (London) 1960;188:151–152.
126.Wunder CC, Cook RM, Welch RC, Glade R, Fleming BP. Femur bending properties as influenced by gravity: I. ultimate load and moment for 3-G rats. Aviat Space Environ Med 1977;48:339–346.
127.Glucksmann A. Studies of bone mechanics in vitro I-Influence of pressure on orientation of structure. Anat Rec 1938;72:97–115.
128.Goodship AE, Lanyon LE, McFie M. Functional adaptation of bone to increased stress. J Bone Jnt Surg 1979;61A:539– 546.
129.Hall BK. Developmental and cellular skeletal biology. New York: Academic Press, 1978.
130.Sumner-Smith G. Bone in clinical orthopaedics. New York: W. B. Saunders; 1982.
131.Lanyon LE, Magee PT, Bagott DG. The relationship of the functional stress and strain to the process of bone remodelling: an experimental study on the sheep radius. J Biomech 1979;12:593–600.
132.Woo SLY, Kuei SC, Amiel D, Gomez MA, Hayes WC, White FC, Akeson WH. The effect of prolonged physical training on the properties of long bone: a study of Wolff’s law. J Bone Jnt Surg 1981;63A:780–787.
133.Liskova M, Hert J. Reaction of bone to mechanical stimuli, part 2, periosteal and endosteal reaction of the tibial diaphysis in rabbit to intermittent loading. Folia Morpholog 1971;19:310–317.
134.Wright KWJ, Yettram AL. An analytical investigation into possible mechanical causes of bone remodelling. J Biomed Eng (England) 1979;1:41–49.
135.Frost HM. Osteocyte death in vivo. J Bone Jnt Surg 1960;42A:138–143.
136.Cowin SC, Hegedus DH. Bone Remodeling I: Theory of adaptive elasticity. J Elasticity 1976;6:313–326.
137.Bassett CAL, Becker RO. Generation of electric potentials in bone in response to mechanical stress. Science 1962;137: 1063–1064.
138.Spadaro JA. Electrically stimulated bone growth in animals and man. Clin Orthopaed 1977;122:325–332.
139.Tischendorf F. Das Verhalten der Haversschen Systeme bei Belastung. Arch Entwicklungsmech Org 1951;145:318–332.
140.Piekarski K, Munro M. Transport mechanism operating between blood supply and osteocytes in long bones. Nature (London) 1977;269:80–82.
141.Jendrucko RJ, Hyman WA, Newell PH, Chakraborty BK. Theoretical evidence for the generation of high pressure in bone cells. J Biomech 1976;9:87–91.
142.Otter T, Salman ED. Hydrostatic pressure reversibly blocks membrane control of ciliary motion in paramecium. Science 1979;206:358–361.
143.Lakes RS, Katz JL. Viscoelastic properties and behavior of cortical bone, Part II, relaxation mechanisms. J Biomech 1979;12:689–698.
BONE AND TEETH, PROPERTIES OF |
539 |
144.Justus R, Luft JH. A mechanicochemical hypothesis for bone remodelling induced by mechanical stress. Calc Tiss Res 1970;5:222–235.
145.Jendrucko RJ. Energetics of hydroxyapatite nucleation in bone, Proc 30th ACEMB, Los Angeles: 1977.
146.Martin RB, Burr DB. A hypothetical mechanism for the stimulation of osteonal remodelling by fatigue damage. J Biomech 1982;15:137–139.
147.Rasmussen H, Bordier P. The Physiological and Cellular Basis of Metabolic Bone Disease. Williams & Wilkins; 1974.
148.Davidovitch Z, Furst L, Shanfield JL, Montgomery PC, Kelischeck S, Laster L, Korostoff E. Biochemical mediators of electrical stimulation of bone cells. 35th ACEMB. Philadelphia: Sept. 1982. p 217.
149.Rodan GA, Bourret LA, Norton LA. DNA synthesis in cartilage cells is stimulated by oscillating electric fields. Science 1978;199:690–692.
150.Sutherland J, Rall M. The relation of adenosine 30 :50 -phos- phate and phosphorylase to the actions of catecholamines and other hormones. Pharmacol Rev 1977;12:265–299.
151.Fukada E, Yasuda I. On the piezoelectric effect of bone. J Phys Soc J 1957;12:1158–1162.
152.Reinish G. Piezoelectric properties of bone as functions of moisture content. Nature (London) 1975;253:626–627.
153.Lakes RS, Katz JL. Dielectric relaxation in cortical bone. J Appl Phys 1977;48:808–811.
154.Burr AJ. Measurements of the dynamic piezoelectric properties of bone as a function of temperature and humidity. J Biomech 1976;1:495–507.
155.Anderson JC, Eriksson C. Electrical properties of wet collagen. Nature (London) 1968;218:167–169.
156.Anderson JC, Eriksson C. Piezoelectric properties of dry and wet bone. Nature (London) 1970;227:491–492.
157.Williams WS. Sources of piezoelectricity in tendon and bone. CRC Crit Rev Bioeng 1974;2:95–117.
158.Lakes RS. The role of gradient effects in the piezoelectricity of bone. IEEE Trans Biomed Eng 1980;BME27:282–283.
159.Korostoff E. Stress generated potentials in bone: relationship to piezoelectricity of collagen. J Biomech 1979;10:41– 44.
160.Korostoff E. A Linear piezoelectric Model for characterizing stress generated potentials in bone. J Biomech 1979;12:335– 347.
161.Gjelsvik A. Bone remodeling and piezoelectricity II. J Biomech 1973;6:187–193.
162.Guzelsu N. A piezoelectric model for dry bone and tissue. J Biomech 1978;11:257–267.
163.Johnson M, Chakkalakal D, Harper RA, Katz JL. Comparison of the electromechanical effects in wet and dry bone. J Biomech 1980;13:437–442.
164.Chakkalakal DA, Johnson MW, Harper RA, Katz JL. Dielectric properties of fluid saturated bone. IEEE Trans Biomed Eng 1980;BME-27:95–100.
165.Athenstaedt H. Permanent electric polarization and pyroelectric behavior of the vertebrate skeleton. VI, the appendicular skeleton of man. Z Anat Entwickl Gesch 1970;131:21–30.
166.Lang SB. Pyroelectric effect in bone and tendon. Nature (London) 1966;212:704–705.
167.Bassett CAL, Pilla AA, Pawluk RJ. A non-operative salvage of surgically resistant pseudarthrosis and non-unions by pulsing electromagnetic fields: a preliminary report. Clinical Orthop 1977;124:128–143.
168.Brighton CT, Friedenberg ZB, Mitchell EI, Booth RE. Treatment of non-union with constant direct current. Clinical Orthop 1977;124:106–123.