
- •VOLUME 1
- •CONTRIBUTOR LIST
- •PREFACE
- •LIST OF ARTICLES
- •ABBREVIATIONS AND ACRONYMS
- •CONVERSION FACTORS AND UNIT SYMBOLS
- •ABLATION.
- •ABSORBABLE BIOMATERIALS.
- •ACRYLIC BONE CEMENT.
- •ACTINOTHERAPY.
- •ADOPTIVE IMMUNOTHERAPY.
- •AFFINITY CHROMATOGRAPHY.
- •ALLOYS, SHAPE MEMORY
- •AMBULATORY MONITORING
- •ANALYTICAL METHODS, AUTOMATED
- •ANALYZER, OXYGEN.
- •ANESTHESIA MACHINES
- •ANESTHESIA MONITORING.
- •ANESTHESIA, COMPUTERS IN
- •ANGER CAMERA
- •ANGIOPLASTY.
- •ANORECTAL MANOMETRY
- •ANTIBODIES, MONOCLONAL.
- •APNEA DETECTION.
- •ARRHYTHMIA, TREATMENT.
- •ARRHYTHMIA ANALYSIS, AUTOMATED
- •ARTERIAL TONOMETRY.
- •ARTIFICIAL BLOOD.
- •ARTIFICIAL HEART.
- •ARTIFICIAL HEART VALVE.
- •ARTIFICIAL HIP JOINTS.
- •ARTIFICIAL LARYNX.
- •ARTIFICIAL PANCREAS.
- •ARTERIES, ELASTIC PROPERTIES OF
- •ASSISTIVE DEVICES FOR THE DISABLED.
- •ATOMIC ABSORPTION SPECTROMETRY.
- •AUDIOMETRY
- •BACTERIAL DETECTION SYSTEMS.
- •BALLOON PUMP.
- •BANKED BLOOD.
- •BAROTRAUMA.
- •BARRIER CONTRACEPTIVE DEVICES.
- •BIOCERAMICS.
- •BIOCOMPATIBILITY OF MATERIALS
- •BIOELECTRODES
- •BIOFEEDBACK
- •BIOHEAT TRANSFER
- •BIOIMPEDANCE IN CARDIOVASCULAR MEDICINE
- •BIOINFORMATICS
- •BIOLOGIC THERAPY.
- •BIOMAGNETISM
- •BIOMATERIALS, ABSORBABLE
- •BIOMATERIALS: AN OVERVIEW
- •BIOMATERIALS: BIOCERAMICS
- •BIOMATERIALS: CARBON
- •BIOMATERIALS CORROSION AND WEAR OF
- •BIOMATERIALS FOR DENTISTRY
- •BIOMATERIALS, POLYMERS
- •BIOMATERIALS, SURFACE PROPERTIES OF
- •BIOMATERIALS, TESTING AND STRUCTURAL PROPERTIES OF
- •BIOMATERIALS: TISSUE-ENGINEERING AND SCAFFOLDS
- •BIOMECHANICS OF EXERCISE FITNESS
- •BIOMECHANICS OF JOINTS.
- •BIOMECHANICS OF SCOLIOSIS.
- •BIOMECHANICS OF SKIN.
- •BIOMECHANICS OF THE HUMAN SPINE.
- •BIOMECHANICS OF TOOTH AND JAW.
- •BIOMEDICAL ENGINEERING EDUCATION
- •BIOSURFACE ENGINEERING
- •BIOSENSORS.
- •BIOTELEMETRY
- •BIRTH CONTROL.
- •BLEEDING, GASTROINTESTINAL.
- •BLADDER DYSFUNCTION, NEUROSTIMULATION OF
- •BLIND AND VISUALLY IMPAIRED, ASSISTIVE TECHNOLOGY FOR
- •BLOOD BANKING.
- •BLOOD CELL COUNTERS.
- •BLOOD COLLECTION AND PROCESSING
- •BLOOD FLOW.
- •BLOOD GAS MEASUREMENTS
- •BLOOD PRESSURE MEASUREMENT
- •BLOOD PRESSURE, AUTOMATIC CONTROL OF
- •BLOOD RHEOLOGY
- •BLOOD, ARTIFICIAL
- •BONDING, ENAMEL.
- •BONE AND TEETH, PROPERTIES OF
- •BONE CEMENT, ACRYLIC
- •BONE DENSITY MEASUREMENT
- •BORON NEUTRON CAPTURE THERAPY
- •BRACHYTHERAPY, HIGH DOSAGE RATE
- •BRACHYTHERAPY, INTRAVASCULAR
- •BRAIN ELECTRICAL ACTIVITY.
- •BURN WOUND COVERINGS.
- •BYPASS, CORONARY.
- •BYPASS, CARDIOPULMONARY.
Control Mechanisms. Vol. II. Portland (OR): University of Oregon Books; 1992. p 216–219.
50.Panzer V, Kaye J, Edner A, Holme L. Standing postural control in the elderly and v elderly. In: Woollacott M, Horak F, editors. Posture and Gait: Control Mechanisms. Vol. II. Portland (OR): University of Oregon Books; 1992. p 220–223.
51.Craik R. Changes in locomotion in the aging adult. In: Woollacott MH, Shumway-Co A, editors. Development of Posture and Gait across the Life Span. Columbia (SC): University of South Carolina Press; 1989. p 150–153.
52.Williams K. Intralimb coordination of older adults during locomotion: Stair climbing. In: Woollacott M, Horak F, editors. Posture and Gait: Control Mechanisms. Vol. II. Portland (OR): University of Oregon Books; 1992. p 208–211.
53.Light KE, Tang PF, Krugh CR. Performance differences between young and elderly females in a step-reach task. In: Woollacott M, Horak F, editors. Posture and Gait: Control Mechanisms. Vol. II. Portland (OR): University of Oregon Books; 1992. p 287–290.
54.Jacobs I, Bell DG, Pope J. Comparison of isokinetic and isoinertial lifting tests as predictors of maximal lifting capacity. Eur J Appl Physiol 1988;57:146–153.
55.Ariel GB. Computerized dynamic resistive exercise. In: Landry F, Orban WAR, editors. Mechanics of Sports and Kinanthropometry. Book 6. Miami (FL): Symposia Specialists, Inc.; 1978. p 45–51.
56.Benson H. University Physics. New York: John Wiley & Sons; 1991.
57.Eddington A. The Nature of the Physical World. Cambridge: New Press; 1928.
See also EXERCISE STRESS TESTING; HUMAN SPINE, BIOMECHANICS OF;
JOINTS, BIOMECHANICS OF; LOCOMOTION MEASUREMENT, HUMAN; REHABILITATION AND MUSCLE TESTING.
BIOMECHANICS OF JOINTS. See JOINTS,
BIOMECHANICS OF.
BIOMECHANICS OF SCOLIOSIS. See SCOLIOSIS,
BIOMECHANICS OF.
BIOMECHANICS OF SKIN. See SKIN, BIOMECHANICS
OF.
BIOMECHANICS OF THE HUMAN SPINE. See
HUMAN SPINE, BIOMECHANICS OF.
BIOMECHANICS OF TOOTH AND JAW. See
TOOTH AND JAW, BIOMECHANICS OF.
BIOMEDICAL ENGINEERING EDUCATION
PAUL BENKESER
Georgia Institute of Technology
Atlanta, Georgia
INTRODUCTION
Biomedical engineering is that interdisciplinary field of study combining engineering with life sciences and medicine. It is a relatively new field of study that has only recently experienced sufficient maturity to enable it to establish its own identity. Often, this field will be described
BIOMEDICAL ENGINEERING EDUCATION |
403 |
using the term bioengineering. In 1997, the Bioengineering Definition Committee of the National Institutes of Health released the following definition of the field (1): ‘‘Bioengineering integrates physical, chemical, mathematical, and computational sciences and engineering principles to study biology, medicine, behavior, and health. It advances fundamental concepts; creates knowledge from the molecular to the organ systems level; and develops innovative biologics, materials, processes, implants, devices and informatics approaches for the prevention, diagnosis, and treatment of disease, for patient rehabilitation, and for improving health.’’
While many use biomedical engineering and bioengineering interchangeably, it is generally accepted today that bioengineering is a broader field that combines engineering with life sciences, but is not necessarily restricted to just medical applications.
The Biomedical Engineering Society further elaborated on the definition of biomedical engineering as part of a guide on careers in the field. In it is stated (2): ‘‘A Biomedical Engineer uses traditional engineering expertise to analyze and solve problems in biology and medicine, providing an overall enhancement of health care. Students choose the biomedical engineering field to be of service to people, to partake of the excitement of working with living systems, and to apply advanced technology to the complex problems of medical care. The biomedical engineer works with other health care professionals including physicians, nurses, therapists and technicians. Biomedical engineers may be called upon in a wide range of capacities: to design instruments, devices, and software, to bring together knowledge from many technical sources to develop new procedures, or to conduct research needed to solve clinical problems.’’
Educational programs in the field of biomedical engineering had their origins in a handful of specialized graduate training programs in the 1950s focusing primarily on diagnostic and therapeutic devices and instrumentation. By 2004, there were undergraduate and graduate programs in biomedical engineering at 100 universities in the United States. The diversity in the content of undergraduate educational programs that was commonplace in its early years is gradually diminishing as the field has matured. While the current undergraduate programs still maintain their own unique identity, there has been a steady movement toward the definition of a core curriculum in the field.
The purpose of this article is to give the reader some historical perspective on the origins of educational programs in the field, the challenges associated with preparing bachelor-level graduates for careers in the field, and the current state-of-the-art in undergraduate biomedical engineering curriculums.
HISTORY
The first steps toward establishing biomedical engineering as a discipline occurred in the 1950s as several formalized training programs were created. Their establishment was significantly aided by the National Institutes of Health
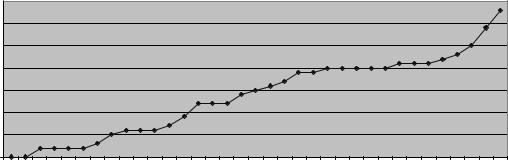
404 BIOMEDICAL ENGINEERING EDUCATION
creation of training grants for doctoral studies in biomedical engineering. The Johns Hopkins University, the University of Pennsylvania, the University of Rochester, and Drexel University were among the first to be awarded these grants.
During the late 1960s and early 1970s, growing opportunities in the field helped prompt the development of a second generation of biomedical engineering programs and departments. These included Boston University in 1966; Case Western Reserve University in 1968; Northwestern University in 1969; Carnegie Mellon University, Duke University, Rensselaer Polytechnic Institute and a joint program between Harvard and the Massachusetts Institute of Technology in 1970; Ohio State University and University of Texas, Austin, in 1971; Louisiana Tech, Texas A&M and the Milwaukee School of Engineering in 1972; and the University of Illinois, Chicago in 1973 (3). Many of these first and second generation of programs were concentrating the training of their students in areas defined either using quasiclassical engineering terminology, such as bioinstrumentation, biomaterials and biomechanics, or by application area, such as rehabilitation engineering or clinical engineering.
The late 1990s witnessed a substantial increase in the growth of the number of departments and programs in biomedical engineering, especially at the undergraduate level. The growth of this third generation of programs was fueled in part by grants from The Whitaker Foundation to help institutions establish or develop biomedical engineering departments or programs. In 2004, 100 universities have programs or departments in biomedical engineering, including 33 offering undergraduate degree programs accredited by the Engineering Accreditation Commission of the Accreditation Board for Engineering and Technology (ABET) (4). The growth in the numbers of ABET accredited degree programs is illustrated in Fig. 1.
The arrival of the third generations of programs coincided with the development of several new areas of training in biomedical engineering, such as systems biology–phy- siology, and tissue, cellular, and biomolecular engineering. These areas typically require significantly more training in life sciences than was present in the first and second
generation biomedical engineering training programs. This presented significant challenges for undergraduate programs trying to add this life science content to their curricula without increasing the number of credit hours required for the programs. Many of these programs accomplished this by creating a new generation of courses in which the engineering and life science concepts are integrated together within courses. The integration of such courses into the curriculum is discussed in more detail in the Curriculum section.
CAREER PREPARATION
The design of a high quality educational program should always start with its educational objectives. By using the definition established by ABET, these program educational objectives are statements that describe the expected accomplishments of graduates during the first several years following graduation (5). This requires programs to be cognizant of the needs of prospective employers of its graduates and design learning environments and curricula to meet those needs. This is particularly challenging task for a relatively new and evolving field like biomedical engineering.
Biomedical engineers are employed in industry, in research facilities of educational and medical institutions, in teaching, in government regulatory agencies, and in hospitals. They often serve as integrators or facilitators, using their skills in both the engineering and life science fields. They may work in teams in industry to help design devices, systems, and processes that require an in-depth understanding of both living systems and engineering. Frequently, biomedical engineers will be found in technical sales and marketing positions in companies seeking to provide their customers with technically trained individuals who are capable of better understanding their needs and communicating those needs back to product development teams. Government regulatory positions, such as those with the Food and Drug Administration, often involve testing medical devices for performance and safety. In research institutions, biomedical engineers participate in or direct research activities in collaboration with other
Programs |
35 |
|
|
|
|
|
|
|
|
|
|
|
|
|
|
|
|
|
30 |
|
|
|
|
|
|
|
|
|
|
|
|
|
|
|
|
|
|
25 |
|
|
|
|
|
|
|
|
|
|
|
|
|
|
|
|
|
|
of Accredited |
|
|
|
|
|
|
|
|
|
|
|
|
|
|
|
|
|
|
20 |
|
|
|
|
|
|
|
|
|
|
|
|
|
|
|
|
|
|
15 |
|
|
|
|
|
|
|
|
|
|
|
|
|
|
|
|
|
|
10 |
|
|
|
|
|
|
|
|
|
|
|
|
|
|
|
|
|
|
Number |
|
|
|
|
|
|
|
|
|
|
|
|
|
|
|
|
|
|
5 |
|
|
|
|
|
|
|
|
|
|
|
|
|
|
|
|
|
|
0 |
|
|
|
|
|
|
|
|
|
|
|
|
|
|
|
|
|
|
|
|
|
|
|
|
|
|
|
|
|
|
|
|
|
|
|
|
|
|
0 |
2 |
4 |
1976 |
1978 |
1980 |
1982 |
1984 |
6 |
8 |
0 |
2 |
4 |
6 |
1998 |
2000 |
2002 |
4 |
|
7 |
7 |
7 |
8 |
8 |
9 |
9 |
9 |
9 |
0 |
||||||||
|
9 |
9 |
19 |
9 |
9 |
9 |
19 |
9 |
19 |
0 |
||||||||
|
1 |
1 |
1 |
1 |
1 |
1 |
2 |
|||||||||||
|
|
|
|
|
|
|
|
|
|
Year |
|
|
|
|
|
|
|
|
Figure 1. Number of ABET accredited programs in biomedical enginering.
researchers with such backgrounds as medicine, biology, chemistry, and a variety of engineering disciplines.
According to the U.S. Department of Labor’s Bureau of Labor Statistics, manufacturing industries employed 38% of all biomedical engineers, primarily in the pharmaceutical and medicine manufacturing and medical instruments and supplies industries (6). Others worked in academia, hospitals, government agencies, or as independent consultants. Employment of biomedical engineers is expected to increase faster than the average for all occupations through 2012 (6). The demand for better and more cost-effective medical devices and equipment designed by biomedical engineers is expected to increase with the aging of the population and the associated increased focus on health issues. Most of these employment opportunities will be filled with graduates from B.S. and M.S. degree programs. However, for research-oriented jobs, like faculty positions in academia and research and development positions in industry, employers typically require their employees to possess a Ph.D. degree.
The needs of the employers that hire biomedical engineers undoubtedly vary by industry and job title. However, there are some skills that appear to be in universal demand by all employers of biomedical engineers. They include proficient oral and written communication skills, the ability to speak the languages of engineering and medicine, a familiarity with physiology and pathophysiology, and teamwork skills (6).
In spite of this seemingly impressive list of career paths and options, one of the most significant challenges facing entry-level biomedical engineers are prospective employers who complain that they do not understand what skill sets biomedical engineers possess (7). The perception is that the skills possessed by engineers from other disciplines, like electrical or mechanical engineering, are more predictable and in large part are independent of the university where the engineer was educated. It is likely that this perception is a result of a combination of two factors. First, often the individuals responsible for making the hiring decisions in companies did not receive their degrees in biomedical engineering, and thus do not have first-hand experience with the training received by biomedical engineers. Second, until relatively recently, many undergraduate biomedical engineering programs lacked a substantive core curriculum and were structured in such a way that students had to select from one of several ‘‘tracks’’ offered by the program. These tracks were typically pattered along traditional engineering lines, such as bioelectronics and biomechanics, in an attempt to address another concern expressed by prospective employers—that graduates of bachelor degree programs in biomedical engineering were too broadly trained and thus lacked sufficient depth of engineering skills. Due to this perceived lack of depth, it is not uncommon to find employers for which the entry-level degree for biomedical engineering positions is the masters degree. Undoubtedly the presence of these tracks, and their variability from program to program, contributed to the confusion in industry over the what skill sets they should expect from a biomedical engineer.
As a result of these concerns over depth, breadth, and uniformity of curriculum, the biomedical engineering
BIOMEDICAL ENGINEERING EDUCATION |
405 |
education community has recognized the need to reach consensus on what constitutes a core undergraduate curriculum in biomedical engineering. This has become one of the major initiatives of the National Science Foundation (NSF) sponsored VaNTH Engineering Research Center (ERC) for Biomedical Engineering Educational Technologies. This ERC, a collaboration of teams from Vanderbilt University, Northwestern University, The University of Texas at Austin, Harvard University, and the Massachusetts Institute of Technology, was created in 1999. Its vision is to transform bioengineering education to produce adaptive experts by developing, implementing and assessing educational processes, materials, and technologies that are readily accessible and widely disseminated (8). The Whitaker Foundation has also sponsored workshops at its 2000 and 2005 Biomedical Engineering Summit meetings with the goal of delineating the core topics in biomedical engineering that all biomedical engineering students should understand. White papers from these meetings can be found at the Foundation’s web site (9).
In spite of the movement toward the creation of a common core curriculum in undergraduate programs of study in biomedical engineering, there will undoubtedly continue to be some differences in curricula between programs. This is not only permitted in the current accreditation review process of ABET, but in some sense encouraged. Within the past decade this process has changed from a prescriptive evaluation to an outcomes-based assessment centered on program-defined missions and objectives (10). Thus, it will be incumbent on programs to work closely with the prospective employers of their graduates to ensure that the programs provide the graduates with the skills the employers desire. For example, Marquette University’s biomedical engineering program has an established industrial partners program with >30 companies participating (11).
THE UNDERGRADUATE CURRICULUM
Contained within this section is a description of a core undergraduate curriculum that the author believes the biomedical engineering educational community is converging upon. The contents are based upon reviews of curriculums from biomedical engineering programs (12) and information disseminated by the Curriculum Project of the VaNTH ERC (13). It is important to note that the core described herein is not being presented as the prescription for what a biomedical engineering curriculum should look like, but rather a reflection of the current trends in the field.
Course Requirements
To be accredited by ABET, the curriculum must include the following:
One year of an appropriate combination of mathematics and basic sciences.
One-half year of humanities and social sciences.
One and one-half years of engineering topics and the requirements listed in ABETs Program Criteria for bioengineering.
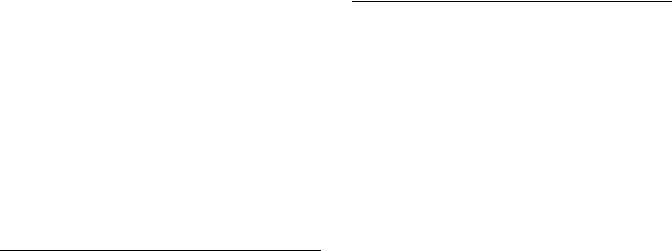
406 BIOMEDICAL ENGINEERING EDUCATION
A year is defined as 32 semester or 48 quarter hours. The typical math and science content of biomedical engineering curriculums, as described in Table 1, are similar to those of other engineering disciplines. The notable exceptions are courses in biology and organic chemistry. In general, most programs rely on other departments within their university to provide the instruction for these courses. Some programs with large enrollments have been successful in collaborating with faculty in their university’s science departments to create biology and chemistry courses for biomedical engineering students. For example, the School of Chemistry and Biochemistry at the Georgia Institute of Technology has created organic and biochemistry courses specifically designed for biomedical engineer-
ing students.
Table 1. Mathematics and Science Core Curriculum
Subjects
Subject |
Sampling of Topical Coverage |
|
|
Math |
Linear algebra |
|
Differential, integral, multivariable |
|
calculus |
|
Differential equations |
|
Statistics |
Physics |
Classical mechanics, oscillations |
|
and waves |
|
Electromagnetism, light and modern |
|
physics |
Computer Science |
Algorithms, data structures, program |
|
design and flow control |
|
Graphics and data visualization |
|
Higher level programming language |
|
(e.g., Matlab, Java, Cþþ) |
Chemistry |
General and inorganic chemistry |
|
Organic chemistry |
|
Biochemistry |
Biology |
Modern biological principles |
|
Genetics |
|
Cell biology |
|
|
The core biomedical engineering content is described in Table 2. Depending on the size of the program, some of the content may be delivered in courses outside of biomedical engineering (e.g., thermodynamics from mechanical engineering). It is not uncommon to find some variability between programs in the content of their core curriculums. This will likely always be the case as each program must provide the curriculum that best enables its graduates to achieve the program’s unique educational objectives.
ABET Criterion 3 stipulates that engineering programs must demonstrate achievement of a minimum set of program outcomes. These outcomes are statements that describe skills that ‘‘students are expected to know or be able to do by the time of graduation from the program’’ (5). A closer examination of these skills suggests that they can be divided into two sets as illustrated in Table 3. The first set, ‘‘domain’’ skills, is one that engineering educators are typically adept in both teaching and quantitatively measuring achievement. Programs generally use courses, like those listed in Tables 1 and 2, to develop these domain
Table 2. Biomedical Engineering Core Curriculum
Subjects
Subject |
Sampling of Topical Coverage |
|
|
Biomechanics |
Principles of statics |
|
Mechanics of biomaterials |
|
Dynamics |
Biotransport |
Mass transfer |
|
Heat transfer |
|
Momentum transfer |
Biothermodynamics |
Thermodynamic principles |
|
Mass and energy balances |
Biomaterials |
Metals, polymers and composite |
|
materials |
|
Biocompatibility |
Bioinstrumentation |
Instrumentation concepts |
|
Amplifiers and filters |
|
Sensors and transducers |
Biofluids |
Blood vessel mechanics |
|
Hydrostatics and steady flow models |
|
Unsteady Flow and non-uniform |
|
geometric models |
Systems Physiology |
Cellular metabolism |
|
Membrane dynamics |
|
Homeostatis |
|
Endocrine, cardiovascular and |
|
nervous systems |
|
Muscles |
Biosignal Analysis |
Digital signal processing theory |
|
Filtering |
|
Frequency-domain characterization |
|
of signals |
|
|
skills in their students. The second set, ‘‘professional’’ skills, is more difficult to teach and assess. However, these professional skills are often the ones most frequently cited by employers of engineers as the most important skills they value in their employees.
Humanities and social science courses are integral to the achievement of these professional skills. However, programs must avoid employing the ‘‘inoculation’’ model for teaching these skills to the students. In this model, it is assumed that students can learn these skills by simply taking isolated courses in ethics, technical communications, and so on. There are several problems with this model. It can decontextualize these skills, treating them as add-ons and not an integral part of everyday engineering practice. This is a false and even dangerous message to give the students—that written and oral communication and ethical behavior are peripheral to the real world of engineering. This message is further driven home because the faculty responsible for teaching these skills is humanities or social science faculty not engineering faculty. In addition, the complexity of these skills to be learned is too great for students to master within the framework of isolated courses. Research suggests, however, that students need quasirepetitive activity cycles and practice in multiple settings to develop proficiency in these professional skills (14–16).
Professional Skills
Before describing methods of developing these professional skills in students, it is necessary to establish operational
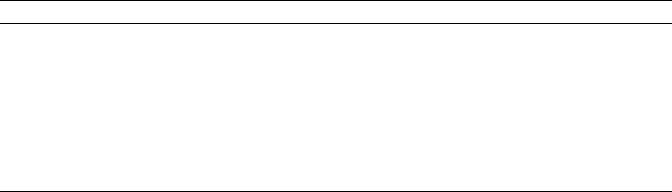
BIOMEDICAL ENGINEERING EDUCATION |
407 |
Table 3. Program Outcomes Specified in ABET Criterion 3
Domain Skills |
Professional Skills |
An ability to apply knowledge of mathematics, science, and engineering
An ability to design and conduct experiments, as well as to analyze and interpret data
An ability to design a system, component, or process to meet desired needs
An ability to identify, formulate, and solve engineering problems
A knowledge of contemporary issues
An ability to use the techniques, skills, and modern engineering tools necessary for engineering practice
An ability to function on multi-disciplinary teams
An understanding of professional and ethical responsibility
An ability to communicate effectively
The broad education necessary to understand the impact of engineering solutions in a global and societal context
A recognition of the need for, and an ability to engage in lifelong learning
descriptions for these constructs. Such descriptions serve two functions. They reveal the complexity of the particular skills in terms of the subskills required to demonstrate the higher level skills specified in the ABET lists. Descriptions can also serve as articulations of learning outcomes, which can be designed toward and assessed. The following represents one interpretation of the variables that are indicators of these constructs (16).
Ability to communicate effectively.
Oral þ written communication skills
Convey information and ideas accurately and efficiently.
Articulate relationships among ideas.
Inform and persuade.
Assemble and Organize evidence in support of an argument.
Make communicative purpose clear.
Provide sufficient background to anchor ideas– information.
Be aware of and address multiple interlocutors.
Clarify conclusions to be drawn from information.
Ability to function on multidisciplinary teams.
Team collaboration skills þ communication skills 3
Help group develop and achieve team goals.
Avoid contributing excessive or irrelevant information.
Confront others directly when necessary.
Demonstrate enthusiasm and involvement.
Monitor group progress and complete tasks on time.
Facilitate interaction with other members.
Understanding professional and ethical responsibilities.
Recognize moral problems and issues in engineering.
Comprehend, clarify, and critically assess opposing arguments.
Form consistent and comprehensive viewpoints based on facts.
Develop imaginative responses to problematic conflicts.
Think clearly in the midst of uncertainty and ambiguity.
Appreciate the role of rationale dialogue in resolving moral conflicts.
Ability to maintain moral integrity in face of pressures to separate professional and personal convictions.
Broad education necessary to understand the impact of engineering solutions in a global and societal context.
Identify human needs or goals technology will serve.
Analyze and evaluate the impact of new technologies on economy, environment, physical and mental health of manufacturers, uses of power, equality, democracy, access to information and participation, civil liberties, privacy, crime and justice.
Identify unintended consequences of technology development.
Create safeguards to minimize problems.
Apply lessons from earlier technologies and experiences of other countries.
Recognition of the need for, and an ability to engage in life-long learning.
Identify learning needs and set specific learning objectives.
Make a plan to address these objectives.
Evaluate inquiry.
Assess the reliability of sources.
Evaluate how the sources contribute to knowledge.
Question the adequacy and appropriateness of forms of evidence used to report back on learning needs.
Apply knowledge discovered to the problem.
408 |
BIOMEDICAL ENGINEERING EDUCATION |
|
|
|
|
|
Table 4. Repetitive Activities in the Problem-Solving Cycle |
|
|
|
|
||
|
|
|
|
|
|
|
|
|
|
|
Professional Skill |
|
|
|
|
|
|
|
|
|
Activity |
|
Communicate |
Teams |
Responsibilities |
Impact |
Learning |
|
|
|
|
|
|
|
Identifying learning–knowledge needs as a team–individual |
|
|
|
|
X |
|
Acquiring knowledge needed to solve problem |
|
|
X |
X |
X |
|
Reporting back to team |
X |
X |
|
|
X |
|
Digging deeper and solving |
X |
X |
X |
X |
X |
|
Presenting solution to audience of experts |
X |
|
|
|
|
|
Writing a report on problem solution |
X |
|
|
|
|
|
|
|
|
|
|
|
|
There are a variety of methods programs can employ to foster the development of these professional skills in students. These include the use of team-based capstone design experiences, facilitating student participation in coop and internship experiences, encouraging involvement in undergraduate research projects, and incorporating oral and written communication exercises throughout the curriculum.
The creation of new undergraduate biomedical engineering programs has led to the development of some new approaches to professional skills development. For example, the Wallace H. Coulter Department of Biomedical Engineering at Georgia Tech and Emory University has implemented an integrative approach to the development of professional skills and adaptive expertise in its program. This approach anchors professional skills development in the context of team-based problem solving and design experiences over the four-year curriculum. This approach provides multiple opportunities for the students to work on and develop skills and knowledge in a variety of ‘‘realworld’’ engineering settings in which these professional skills are practiced. Within each experience, activities are identified within the problem-solving cycle that help students develop these professional skills. These activities are described in Table 4.
The need for real-world problems cannot be overstated. Authentic, open-ended problems are needed as contexts and catalysts for the development of these professional skills. Not only do they help prepare students for the professional practice of engineering, but they are also a significant motivator for the students to delve more deeply into the problem space. Moreover, the use of these skills in the context of a large problem makes them central not peripheral to biomedical engineering problem solving. They begin to understand the value of clear, thoughtful communication, and collaboration when confronting complex problems. They see how ethical issues can arise when seeking design solutions. If the problems are authentic, then the information needed to solve them must be found in multiple places, which helps students to develop inquiry and research skills for lifelong learning.
SUMMARY
The field of biomedical engineering had its foundations laid roughly 50 years ago. Undergraduate degree programs in the field followed shortly thereafter. Fueled in part by generous support from the Whitaker Foundation, there has been a significant increase in the number of new
undergraduate degree programs in the field. This has led to a significant increase in student interest in the field. This growth has increased the need for the biomedical engineering education community to work with industry to better define the skills that graduates need to obtain to lead productive careers in the field. There exists a movement, led by the NSF VaNTH ERC, to define a core undergraduate curriculum within the constraints imposed by ABET accreditation criteria. The VaNTHs vision to transform bioengineering education to produce adaptive experts has been adopted by new undergraduate degree programs and has produced demonstrated examples of pedagogical advances in the field of engineering education.
BIBLIOGRAPHY
Cited References
1.NIH working definition of bioengineering. 1997, July 24. National Institutes of Health Bioengineering Consortium. Available at http://www.becon.nih.gov/bioengineering_definition.htm. Accessed 2004 Nov. 18.
2.Planning a career in biomedical engineering. 1999. Biomedical Engineering Society. Available at http://www.bme- s.org/careers.asp. Accessed 2004 Nov. 18.
3.A history of biomedical engineering. 2002, May. The Whitaker Foundation. Available at http://www.whitaker.org/ glance/definition.html. Accessed 2004 Nov. 19.
4.Accredited engineering programs. 2004. Accreditation Board for Engineering and Technology. Available at http://www. abet.org/ accredited_programs/engineering/EACWebsite.asp. Accessed 2004 Nov. 19.
5.Criteria for accrediting engineering programs. 2004. Accreditation Board for Engineering and Technology. Available at http://www.abet.org/criteria.html. Accessed 2004 Nov. 19.
6.Bureau of Labor Statistics, U.S. Department of Labor, Occupational Outlook Handbook. 2004–2005 edition, Biomedical Engineers. Available at http://www.bls.gov/oco/ocos262.htm. Accessed 2005 Feb. 10.
7.RA Linsenmeier, What makes a biomedical engineer, IEEE Eng Med Biol Mag 2003;22(4):32–38.
8.Cordray DS, Pion GM, Harris A, Norris P. The value of the VaNTH Engineering Research Center. IEEE Eng Med Biol Mag 2003;22(4):47–54.
9.Biomedical Engineering Educational Summit, The Whitaker Foundation. Available at http://summit.whitaker.org/. Accessed 2005 Feb 10.
10.Enderle J, Gassert J, Blanchard S, King P, Beasley D, Hale P, Aldridge D. The ABCs of preparing for ABET. IEEE Eng Med Biol Mag 2003;22(4):122–132.