
- •VOLUME 3
- •CONTRIBUTOR LIST
- •PREFACE
- •LIST OF ARTICLES
- •ABBREVIATIONS AND ACRONYMS
- •CONVERSION FACTORS AND UNIT SYMBOLS
- •EDUCATION, COMPUTERS IN.
- •ELECTROANALGESIA, SYSTEMIC
- •ELECTROCARDIOGRAPHY, COMPUTERS IN
- •ELECTROCONVULSIVE THERAPHY
- •ELECTRODES.
- •ELECTROENCEPHALOGRAPHY
- •ELECTROGASTROGRAM
- •ELECTROMAGNETIC FLOWMETER.
- •ELECTROMYOGRAPHY
- •ELECTRON MICROSCOPY.
- •ELECTRONEUROGRAPHY
- •ELECTROPHORESIS
- •ELECTROPHYSIOLOGY
- •ELECTRORETINOGRAPHY
- •ELECTROSHOCK THERAPY.
- •ELECTROSTIMULATION OF SPINAL CORD.
- •ELECTROSURGICAL UNIT (ESU)
- •EMERGENCY MEDICAL CARE.
- •ENDOSCOPES
- •ENGINEERED TISSUE
- •ENVIRONMENTAL CONTROL
- •EQUIPMENT ACQUISITION
- •EQUIPMENT MAINTENANCE, BIOMEDICAL
- •ERGONOMICS.
- •ESOPHAGEAL MANOMETRY
- •EVENT-RELATED POTENTIALS.
- •EVOKED POTENTIALS
- •EXERCISE FITNESS, BIOMECHANICS OF.
- •EXERCISE, THERAPEUTIC.
- •EXERCISE STRESS TESTING
- •EYE MOVEMENT, MEASUREMENT TECHNIQUES FOR
- •FETAL MONITORING
- •FETAL SURGERY.
- •FEVER THERAPY.
- •FIBER OPTICS IN MEDICINE
- •FICK TECHNIQUE.
- •FITNESS TECHNOLOGY.
- •FIXATION OF ORTHOPEDIC PROSTHESES.
- •FLAME ATOMIC EMISSON SPECTROMETRY AND ATOMIC ABSORPTION SPECTROMETRY
- •FLAME PHOTOMETRY.
- •FLOWMETERS
- •FLOWMETERS, RESPIRATORY.
- •FLUORESCENCE MEASUREMENTS
- •FLUORESCENCE MICROSCOPY.
- •FLUORESCENCE SPECTROSCOPY.
- •FLUORIMETRY.
- •FRACTURE, ELECTRICAL TREATMENT OF.
- •FUNCTIONAL ELECTRICAL STIMULATION
- •GAMMA CAMERA.
- •GAMMA KNIFE
- •GAS AND VACUUM SYSTEMS, CENTRALLY PIPED MEDICAL
- •GAS EXCHANGE.
- •GASTROINTESTINAL HEMORRHAGE
- •GEL FILTRATION CHROMATOGRAPHY.
- •GLUCOSE SENSORS
- •HBO THERAPY.
- •HEARING IMPAIRMENT.
- •HEART RATE, FETAL, MONITORING OF.
- •HEART VALVE PROSTHESES
- •HEART VALVE PROSTHESES, IN VITRO FLOW DYNAMICS OF
- •HEART VALVES, PROSTHETIC
- •HEART VIBRATION.
- •HEART, ARTIFICIAL
- •HEART–LUNG MACHINES
- •HEAT AND COLD, THERAPEUTIC
- •HEAVY ION RADIOTHERAPY.
- •HEMODYNAMICS
- •HEMODYNAMIC MONITORING.
- •HIGH FREQUENCY VENTILATION
- •HIP JOINTS, ARTIFICIAL
- •HIP REPLACEMENT, TOTAL.
- •HOLTER MONITORING.
- •HOME HEALTH CARE DEVICES
- •HOSPITAL SAFETY PROGRAM.
- •HUMAN FACTORS IN MEDICAL DEVICES
- •HUMAN SPINE, BIOMECHANICS OF
size, shape, composition, and interactions of specific molecules and about distribution of large numbers of molecules at one time.
For clinical purposes, the resolution of isozymes such as lactic dehydrogenase is reliable enough that the technique has been used in critical care diagnoses, that is, life or death decisions.
BIBLIOGRAPHY
Cited References
Note: Developments in this field are covered particularly in the journal Electrophoresis. The catalogues of companies that sell electrophoresis apparatus and supplies, such as Amersham Biosciences, BioRad, Invitrogen, and National Diagnostics are another sources of information.
1.Tanford C. Physical Chemistry of Macromolecules. New York: John Wiley & Sons; 1961.
2.Brewer JM. Electrophoresis. In: Kaplan LA, Pesce AJ, Kazmierczak SC, editors. Clinical Chemistry: Theory, Analysis, Correlation. 4th ed. St. Louis (MO): Mosby; 2003. Chapt. 10, p 201–215.
3.Cantor CR, Schimmel PR. Biophysical Chemistry. San Francisco: W.H. Freeman and Company; 1980.
4.Moore WJ. Physical Chemistry. 4th ed. Englewood Cliffs (NJ): Prentice-Hall; 1972.
5.Kiriukhin MY, Collins KD. Dynamic hydration numbers for biologically important ions. Biophys Chem 2002; 99:155–168.
6.Rupley JA, Gratton E, Careri G. Water and globular proteins. Trends Biochem Sci 1983;8:18–22.
7.Mosher RA, Saville DA, Thormann W. The Dynamics of Electrophoresis. Weinheim, Germany: VCH; 1992.
8.Marshall AG. Biophysical Chemistry: Principles, Techniques and Applications. New York: John Wiley & Sons; 1978.
9.Righetti PG. Immobilized pH gradients, theory and methodology. Amsterdam, The Netherlands: Elsevier; 1990.
10.Hawcroft DM. Electrophoresis: the basics. Oxford (UK): Oxford University Press; 1997.
11.Whatley H. Basic Principles and Modes of Capillary Electrophoresis. In: Petersen JR, Mohammad AA, editors. Clinical and Forensic Applications of Capillary Electrophoresis. Totowa, NJ: Humana Press; 2001. Chapt. 2, p 21–58.
12.Van Holde KE. Physical Biochemistry. 2nd ed. Engelwood Cliffs (NJ): Prentice-Hall; 1985.
13.Dunn MJ. Gel Electrophoresis: Proteins. Oxford (UK): Bios Scientific; 1993.
14.Noolandi J. Theory of DNA gel electrophoresis. In: Chrambach A, Dunn MJ, Radola BJ, editors. Volume 5, Advances in Electrophoresis. New York: VCH; 1992. p 1–57.
15.Gallagher SR, Winston SE, Fuller SA, Harrell JGR. Immunoblotting and immunodetection. In: Ausubel FM, Brent R, Kingston RE, Moore DD, Seidman JG, Smith JA, Struhl K, editors. Current protocols in molecular biology. Unit 10.8, New York: Greene Publishing and Wiley Interscience; 2000.
16.Baldo BA. Protein blotting: Research, Applications and its place in protein separation methodology. In: Chrambach A, Dunn MJ, Radola BJ, editors. Advances in Electrophoresis. Volume 7, New York: VCH; 1994. p 409–478.
17.Highsmith WE, Jr., Constantine NT, Friedman KJ. Molecular Diagnostics. In: Kaplan LA, Pesce AJ, Kazmierczak SC, editors. Clinical Chemistry: Theory, Analysis, Correlation. 4th ed. St. Louis (MO): Mosby; 2003. Chapt. 48, p 937– 959.
ELECTROPHYSIOLOGY 141
18.Hochstrasser DF, Tissot JD. Clinical application of highresolution two-dimensional gel electrophoresis. In: Chrambach A, Dunn MJ, Radola BJ, editors. Advances in Electrophoresis. Volume 6, New York: VCH; 1993. p 270–375.
19.Gorg A, Obermaier C, Boguth G, Harder A, Scheibe B, Wildgruber R, Weiss W. The current state of twodimensional electrophoresis with immobilized pH gradients. Electrophoresis 2000;21:1037–1053.
20.Merril CR. Gel-staining techniques. Volume 182, Methods Enzymology. New York: Academic Press; 1990. p 477–488.
21.Heeb MJ, Gabriel O. Enzyme localization in gels. Volume 104, Methods Enzymology. New York: Academic Press; 1984. p 416–439.
22.Sanger F, Nicklen S, Coulson AR. DNA sequencing with chain-terminating inhibitors. Proc Natl Acad Sci USA 1977;74:5463–5467.
See also CHROMATOGRAPHY; DNA SEQUENCE.
ELECTROPHYSIOLOGY
SEMAHAT S. DEMIR
The University of Memphis and
The University of Tennessee
Memphis, Tennessee
INTRODUCTION
The Resting Membrane Potential
The cell membrane or sarcolemma, composed of lipid bilayer, is hydrophobic and is highly impermeable to most water-soluble molecules and ions. A potential is developed across the lipid bilayer of the cell membrane due to unequal distribution of charges on the two sides of the membrane, thus the membrane acts as a capacitor. Membrane proteins that span across cell membranes form ion channels allowing transport of small water-soluble ions across the membrane. These channels are highly selective and their selectivity depends on diameter, shape of the ion channel, on the distribution of charged amino acids in its lining (1). The movements of the ions through these channels across the membrane govern its potential.
The transport of the ions across the membrane is either passive or active. The passive mechanism of transport of any ion is governed by its electrochemical gradient, a combination of chemical force exerted by diffusion of ions due to concentration gradient and an electrical force exerted by the electric field developed due the charges accumulated on either side of the membrane (capacitor)
(2). Physiologically, cells have a high intracellular potassium concentration, [Kþ]i, and a low sodium concentration, [Naþ]i. Conversely, the extracellular medium is high in Naþ and low in Kþ. In cardiac cells at rest, the membrane is mostly permeable to Kþ ions through Kþ leak channels. As Kþ flows out down its concentration gradient, a negative potential is built up inside the cell. This increases as long as it counterbalances the chemical driving force generated by concentration gradient. This potential at which the net ion flux is zero is called Nernst equilibrium potential of that ion. The equilibrium potential for Kþ is given by its

142 ELECTROPHYSIOLOGY
Nernst equation:
EK ¼ RT ln ½Kþ&o
ZF ½Kþ&i
where R is the universal gas constant, T is temperature in kelvin, F is Faraday constant, z is the valency of the ion.
Typical resting membrane potentials of excitable cells vary from 90 to 50 mV, depending on the type of the cell. Epithelial cell and erythrocytes have smaller, but still negative membrane potentials. It may tend toward the excitatory threshold for an action potential as in a cardiac pacemaker cell or remain stable with approximately no net ion flux observed in nonpaced cardiac ventricular cells. As the ventricular cell at rest is more permeable to Kþ ions than to any other ion, the resting membrane potential (ca. 84 mV) is close to EK at 37 8C. Due to its permeability to other ions and also due to other transport mechanisms the resting membrane potential does not reach exactly EK.
The active ionic transport mechanisms maintain the homeostasis of ionic concentrations in both the intraand extracellular media. These membrane proteins are called carrier (pump) proteins and they utilize energy from hydrolysis of adenosine triphosphate (ATP) to transport ions against their concentration gradient.
EXPERIMENTAL TECHNIQUES TO QUANTIFY IONIC MECHANISMS IN CELLS
The advent of patch clamp technique (3–7) has made it possible to record the current from a single ion channel. The technique involves clamping a patch of the cell membrane and recording either voltage (current–clamp) or current (voltage–clamp or patch–clamp) across the membrane. Using this technique current of order as low as 10 12 A can be measured. This could be done using different configurations of patch clamping (7).
1. A freshly made glass pipette with a tip diameter of only a few micrometers is pressed gently on the cell membrane to form a gigohm seal. This is called as cell-attached patch configuration. The pipette solutions form the extracellular solution and the currents across the channel within the patch can be recorded.
2. When gentle suction is applied to the pipette in cellattached configuration, the membrane ruptures while maintaining the tight seal and the cytoplasm and pipette solution start to mix. After a short time, this mixing is complete and the ionic environment in the cell is similar to the filling solution used in the pipette. This configuration is called whole-cell patch configuration. A recording obtained using this configuration is from whole cell and not from a patch. The advantage of this technique is that the intracellular environment is accessible through the pipette. Current–clamp technique is used in this configuration to measure the action potentials (APs) of excitable cells.
3. Sudden pulling out of the pipette from cell-attached configuration holds the patch that formed the gigohm seal giving raise to the inside–out configuration (inside of the cell membrane is exposed to external bath).
4. Slow pulling out of the pipette from whole cell configuration holds the patch that formed the gig-ohm seal giving rise to outside–out configuration. Both inside–out and outside–out configurations allow single channel recordings. Both the intracellular and extracellular baths are accessible in these cases.
5. The fifth configuration is obtained by creating artificial channels (permealizing membrane) on the cell-attached patch by administering antibiotics, like amphotericin. The voltage and current–clamp- ing recordings obtained in this configuration recordings are similar to whole-cell recordings, the advantage being the intracellular medium is not dialyzed.
VOLTAGE-CLAMP TECHNIQUE
The method of voltage clamping has been the primary experimental tool used to reconstruct models of cellular electrical activity. As the behavior of the ion channels is highly nonlinear under changing action potential, this method enables us to quantify their properties by holding the transmembrane potential (membrane potential) at a particular voltage. The basic principle relies on providing current to balance those currents through the ionic channels that are open and thus the transmembrane voltage is clamped at a chosen constant level (clamp voltage) For example, if the Naþ channel is studied, the membrane potential is initially held at rest. When this potential is changed instantaneously to a depolarized (more positive) potential, sodium channels open and Naþ ions tends to move in. The voltage amplifier senses these small changes in voltage and a feedback current of equivalent amount is applied in opposite direction of the ion flow. This measurable current changes for different clamp potentials as the
driving force (VClamp–ENa), and the gating parameters at that VClamp changes enabling us to quantify the channel properties. Ion channels conduct ions at a rate sufficiently
high that the flux through a single channel can be detected electrically using patch clamp technique. The basic circuit of voltage clamp setup is shown in Fig. 1.
CURRENT–CLAMP TECHNIQUE
The current–clamp technique is used to record action potentials. This technique involves clamping the cell in whole cell configuration and applying a suprathreshold current pulse for a short duration until the Naþ channels start to activate. The transmembrane change in voltage gives the action potential recording.
A key concept to modeling of excitable cells is the idea of ion channel selectivity of the cell membrane. As the molecular behavior of channels is not known, modeling of
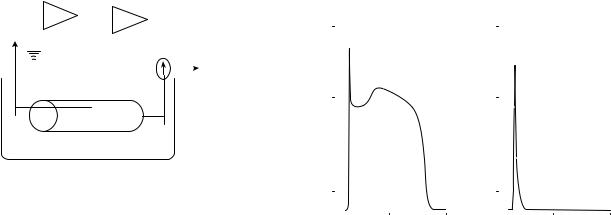
|
|
|
|
Vm |
(VClamp-Vm) |
||||||
Measured |
|
|
|
|
|
|
|
|
|
|
|
|
|
|
|
|
|
|
|
|
|
||
voltage |
|
|
|
|
|
|
|
|
|
||
|
|
VClamp |
|
|
|
Feedback |
|||||
|
|
|
|
|
|
|
|
||||
|
|
|
|
|
|
|
|||||
|
|
|
|
|
|
|
|
|
|
|
current |
|
|
|
|
|
|
|
|
|
|
|
generator |
Cell
Extracellular medium
Figure 1. Simplified circuit representation of a voltage–clamp setup (8).
nonlinear empirical models of membrane processes help us to understand the role of various currents in the depolarization and repolarization phases and the phenomena that involve the interaction between these processes.
EXAMPLE OF ACTION POTENTIAL AND UNDERLYING IONIC BASIS: THE CARDIAC ACTION POTENTIAL
Membrane excitability is the fundamental property of the nerve and muscle cells, that is, in response to certain environmental stimuli, generates an all-or-none electrical signal or AP. Many different types of cardiac ion channels and ion pumps altogether form a complex process that results in cardiac AP (9). For example, the normal cardiac action potentials can be classified into two broad categories; those that are self-oscillatory in nature, such as pacemaker cells (sinoatrial and atrioventrciular cells) and those that need an external stimulus above a threshold, also called supra threshold, in order to be evoked, such as atrial, Purkinjee fiber, or ventricular cells. An extraordinary diversity in the action potential configurations can be seen in different regions of the heart. The ventricular tissue in particular displays a wide variety of action potential waveforms. These APs include pacemaker potentials in purkinje cells, and disparate action potential durations (APD) and morphologies in cells from the epicardial, mid-myocardial, and the endocardial layers of the ventricle. The ventricular action potential has been studied more frequently than other representative cardiac membrane potentials because ventricular arrhythmias are believed to constitute the majority of reportedly fatal incidences of cardiac arrhythmias (10).
Phases of Cardiac Action Potential
A typical ventricular action potential in higher mammals such as canine and human consists of four distinct phases (Fig. 1a). Phase 0 corresponds to a rapid depolarization or upstroke of the membrane action potential. Phase 1 is the initial rapid repolarization, and is followed by phase 2, which constitutes the action potential plateau. Phase 3 represents the final repolarization, which allows the ventricular cell to return to its resting state in phase 4. In addition to its morphological features, ventricular APs are commonly measured experimentally to determine its char-
ELECTROPHYSIOLOGY 143
Examples of Simulated Cardiac Venticular Action Potentials
|
60 |
|
Dog |
|
|
60 |
|
|
Rat |
|
|
|
|
|
|
|
|
|
|||||
|
|
|
|
|
|
|
|
|
|
||
|
|
|
Phase 1 |
|
|
|
|
|
Phase 1 |
|
|
(mV) |
|
|
|
|
|
|
|
|
|
|
|
0 |
|
Phase 2 |
|
|
0 |
|
|
|
|
|
|
Voltage |
|
|
|
|
|
|
|
|
|||
|
|
Phase 0 |
|
|
|
|
|
|
|
|
|
|
|
|
|
|
|
|
|
|
|
|
|
|
|
|
|
|
Phase |
|
0 |
|
|
|
|
|
|
|
Phase 3 |
|
|
|
|
|
Phase 3 |
|
|
|
|
|
|
|
|
|
|
|
|
|
|
|
–80 |
|
|
Phase 4 |
–80 |
|
|
|
Phase 4 |
||
|
|
|
|
|
|
|
|
||||
|
|
|
|
|
|
|
|
|
|
|
|
|
0 |
250 |
500 |
0 |
250 |
500 |
|||||
|
|
|
Time (ms) |
|
|
|
|
|
Time (ms) |
||
|
|
|
(a) |
|
|
|
|
|
(b) |
|
|
Figure 2. Examples of typical cardiac action potential waveforms of the dog (11) and rat (12) ventricular cell models. The action potential of the human ventricular cell would be similar to that of the dog (panel a). Please consult Tables 1 and 2 for the underlying ionic currents.
acteristics. These include the resting membrane potential
(Vrest), the peak overshoot (PO) value that is the maximum positive value achieved during the initial phase 0 depolar-
ization, the maximum upstroke velocity (dV/dtmax), which occurs during phase 0, and the APDs measured when the APs have repolarized to 50 and 90% of their final repolarization value, also called APD50 and APD90, respectively.
One or more of these characteristics is usually altered in the setting of a pathophysiological condition, and helps to quantify the differences between the normal and the abnormal action potentials.
Contributions of Ion Channels to Cardiac Action Potential
The temporal changes in a typical ventricular action potential configuration, that is, depolarization followed by a repolarization (Fig. 2) are governed by the movement of different ions, such as Naþ, Kþ, and Ca2þ ions across the sarcolemma. These ions are usually transported between the intracellular and the extracellular spaces by means of carrier proteins and channel proteins embedded in the cardiac membrane. These proteins form the passive (ion channel-mediated and carrier-mediated) and active (carrier proteins such as pumps and exchanger) transporters of the cell membrane. The ion channels, the pumps and exchanger are the major ionic currents that form an action potential in mathematical representations. A summary of these currents that are present in a typical cardiac ventricular cell and the role of the currents in action potential generation are summarized in Table 1. The major ionic currents contributing to different phases of the typical action potential are presented in Table 2. The ventricular action potential is the result of a delicate balance of the inward and outward ionic currents and the active transporters (pumps and exchangers).
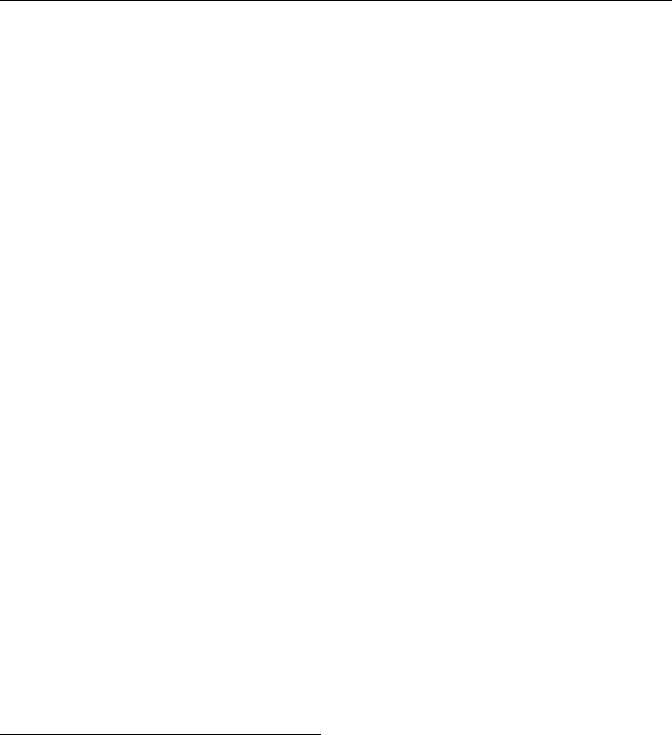
144 ELECTROPHYSIOLOGY
Table 1. Major Ionic Membrane Mechanisms Underlying a Typical Cardiac Ventricular Action Potential
Membrane Mechanism |
Description |
Gene (a-subunit) |
Role in Action Potential |
|
|
|
|
|
Inward Ionic Currents |
|
|
INa |
Naþcurrent |
SCN5A |
Initial depolarization of action potential |
ICaL |
L-type Ca2þ current |
a1C, a1D |
Maintains plateau phase of action potential |
ICaT |
T-type Ca2þ current |
a1G, a1H |
Present in the late plateau phase |
|
Outward Ionic Currents |
|
|
It |
Ca2þ-independent transient |
Kv4.2 |
Responsible for early repolarization |
|
outward Kþ current |
Kv4.3 |
|
|
|
Kv1.4 |
|
IKr,IKs |
Rapid and slow delayed Kþ |
HERG |
Aids repolarization during plateau |
|
rectifier currents |
KvLQT1 |
|
Iss,IKslow |
Slow inactivating Kþ currents |
Kv2.1 |
Aids late repolarization |
|
|
Kv1.5 |
|
IK1 |
Inward rectifier Kþ current |
Kir2.1 |
Late repolarization, helps establish Vrest |
|
|
Kir2.2 |
|
|
Other Ionic Currents |
|
|
INaCa |
Naþ–Ca2þ exchanger current |
NCX1 |
Late depolarization |
|
|
NCX2 |
|
INaK |
Naþ–Kþ pump current |
Naþ-Kþ-ATPase (a) |
Late repolarization |
To restore the intracellular and extracellular ionic concentrations so that homeostasis is maintained, the ions that cross the cell membrane during an action potential are brought back by active mechanisms like Naþ–Kþ pump, Ca2þ pump and coupled transporters like Naþ–Ca2þ exchanger, Naþ–Hþ exchanger. All the active mechanisms utilize hydrolysis of adenosine triphosphate (ATP), the cellular source of energy to achieve this. Of these, Naþ– Kþ pump, which brings in 2 Kþ ions for 3 Naþ ions out per ATP consumed, results in a net positive current (INaK) in outward direction and Naþ–Ca2þ exchanger, which exchanges 3 Naþ ions for one Ca2þ ion, results in a net positive current (INaCa) contributing a little to the action potential. In most species, the exchanger current is in its Ca2þ influx mode (reverse mode) during depolarization resulting in outward current and is inward during Ca2þ efflux mode during repolarization. This is because the equilibrium potential of the current given by (3ENa– 2ECa) is around 40 mV (13). The current contributed by Ca2þ pump is negligible as it pumps few ions across membrane. The Naþ–Hþ exchanger transports one Naþ for Hþ thereby causing no net flux across the membrane.
By convention, any current inward is considered negative and contributes to depolarization and any current outward is considered positive and contributes to repolarization. An inward current of a cation adds positive charge
Table 2. The Action Potential Phases and the Major Ionic Current Contributors
Phases of |
|
Major |
Action |
|
Contributing |
Potentials |
Description |
Ionic Currents |
|
|
|
Phase 0 |
Initial depolarization |
INa |
Phase 1 |
Early repolarization |
It |
Phase 2 |
Plateau phase |
ICaL, IKr, IKs, ICaT |
Phase 3 |
Late repolarization |
IK1, INaK, INaCa,Iss, |
|
|
IKslow, IKr, IKs, |
Phase 4 |
Resting potential |
IK1 |
to the intracellular content, thereby making the transmembrane potential more positive; that is, depolarizes the membrane away from the resting potential.
MATHEMATICAL MODELING OF CARDIAC CELLS
The Hodgkin–Huxley (HH) paradigm (14) type formalism is approached for numerical reconstruction of ventricular AP. At any particular moment, the movement of any particular ion across the membrane depends on the relative density of the channels, the probability of the channel that is selective to the ion being open, the conductance of the ion, and the net driving force of the ion given by the difference (Vm– Eion), where Vm is the transmembrane
voltage and Eion is the Nernst potential of the ion (2). Also, it is assumed that ion fluxes are independent of each other,
that is, the probability of an ion crossing the membrane does not depend on the probability of a different ion crossing the membrane. Based on this, the cell membrane is modeled as a capacitance in parallel to the resistances that represent the flow of ions through their respective channels along with their driving force. The resistive components are characterized in the original HH model as conductance’s (g), the reciprocals of the resistances. The resistive currents can therefore be written
Iion ¼ gion ðVm EionÞ
The experiments suggested that these conductances could be voltage and time dependent resulting in a gating mechanism. In HH type models, this gating mechanism is explained by considering the conductance gion as the product of maximum conductance gion-max the channel can achieve and gating variables whose value lie between 0 and 1. The behavior of a gating variable x is given by first order differential equation:
dx=dt ¼ ðx1 xÞtx
where x1 is the steady-state value the variable reaches at a particular voltage and tx is the time constant at that voltage that determines the rate at which steady state is reached. These variables are voltage dependent. All of these parameters are constrained by experimental data. These models represent the lumped behavior of the channels.
Increased understanding of the behavior of ion channels at a single channel level due to improved patch–clamp techniques lead to the development of state specific Markov models. Based on single channel recordings, it is observed that the channel opening or closing is random. Hence, the conductance gion-max is multiplied by the total open channel probability of the ion (Pion). These models represent the channel behavior based on their conformational changes and are capable of reproducing single channel behavior (15).
The rate of change of membrane potential to a stimulus
current (Ist) is given by
X
ðdV=dtÞ ¼ ð 1=CmÞ |
Ii þ ISt |
where Cm is the membrane capacitance, and Ii values are different ionic currents.
THEORETICAL RESEARCH AND EXPERIMENTAL RESEARCH IN MURINE CARDIAC VENTRICULAR CELLS
After the first models of the mammalian ventricular cells by Beeler and Reuter (16) and Drouhard and Roberge (17), sophisticated mathematical models that simulate the cardiac action potentials in ventricular cells from different species such as canine (18,19), guinea pig (20–24), human (25,26), frog (27), and rabbit (28) have been published during the past decade. The model equations have usually based on the Hodgkin–Huxley (14) paradigm, wherein an ionic current is described by a set of nonlinear differential equations, and the parameters within these equations are constrained by experimental data obtained via voltage– clamp experiments in ventricular myocytes. There is a growing recognition that it is important to understand the complex, nonlinear interactions between the ionic milieu of the cardiac cell, that ultimately influence the action potential (29).
The mathematical models have demonstrated to be useful didactic tools in research, and have also quantified the important functional differences in the action potential properties between different species. Additionally, the computational models have also provided valuable, semiquantitative insights into the diverse ionic mechanisms underlying the normal/abnormal action potential behavior in different animal models. It is not always possible to make precise experimental measurements regarding the contribution of a particular ionic mechanism to an aberrant action potential. The simulation results from these cardiac models have helped in planning for future experimental studies, and also in making predictions in cases where suitable technology is unavailable (or not developed) to make direct experimental measurements (e.g., visualizing the transmural activity within the ventricular wall). These models will play increasingly important roles in
ELECTROPHYSIOLOGY 145
addition to experimental studies in the design and development of future drugs and devices (30). An additional and important feature of these ventricular models has been their ability to simulate intracellular Ca2þ transient ([Ca2þ]i). Thus these models incorporate the feedback mechanism between the APD and the intracellular calcium [Ca2þ]i. The APD is known to influence the amplitude of the [Ca2þ]i in ventricular cells (31), and [Ca2þ]i in turn influences the action potential waveform by Ca2þ-induced Ca2þ inactivation of ICaL, and by determining the peak magni-
tude of INaCa (13).
The previously developed mathematical models of human, dog, guinea pig, rabbit and frog provide a good basis for the understanding of the ionic mechanisms responsible for the generation of the cardiac action potential. However, there are significant differences in the action potential waveforms and their corresponding properties between different species. The unique nature of the rat cardiac action potential, coupled with the recent available experimental data for the ionic mechanisms involved in the genesis of the action potential in isolated rat myocytes, provided the motivation for us to develop the first detailed mathematical model of the rat ventricular action potential. An adult male rat ventricular myocyte model was constructed (32) and utilized this model to study the ionic basis underlying the action potential heterogeneity in the adult rat left ventricle. Important insights into the role of long lasting Ca2þ current (ICaL), the Ca2þ-independent transient outward Kþ current (It), and the steady-state outward Kþ current (Iss) in determining the electrophysiological differences between epicardial and endocardial cells were obtained. This ventricular cell model has been used to investigate the ionic mechanisms that underlie altered electrophysiological characteristics associated with the short-term model of streptozotocin induced, type-I diabetic rats (33) and spontaneously hypertensive rats (34).Our rat ventrcicular myocyte model was further utilized to develop models for the mouse apex and septal left ventricular cells (35–37). Thus these model simulations reproduce a variety of experimental results, and provide quantitative insights into the functioning of ionic mechanisms underlying the regional heterogeneity in the adult rat and mouse ventricle.
The ventricular cell models of dog, guinea pig, human, and rabbit described in the previous section have been mainly used to simulate the so-called spike and dome configurations for action potentials (Fig. 2A) commonly observed in ventricular cells from larger mammalian species (38). However, no mathematical model has been published to represent the murine (rat or mouse) cardiac action potential (Fig. 2B) until our rat ventricular cell (12). The murine ventricular action potentials have a much shorter APD (typically the APD at 90% repolarization (APD90) is < 100 ms), and lack a well-defined plateau phase (triangular in shape) (39–41). A comparison of the experimentally recorded ionic currents underlying action potentials in rat–mouse and other mammalian ventricular cells shows that they display markedly different amplitudes and time-dependent behavior. In fact, despite the similarity of action potential waveforms in rat and mouse, the underlying nature of the repolarizing Kþ currents are
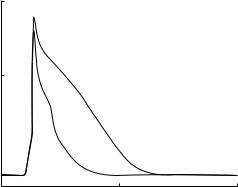
146 ELECTROPHYSIOLOGY
different (41–43). Thus the unique action potential characteristics, and the lack of models to quantify these membrane properties provided the motivation to develop the rat and mouse ventricular cell models. The other motivation in this case was the widespread use of the murine cardiovascular system for the investigation of the cellular and molecular physiology of the compromised cardiovascular function (44).
Experimental studies indicate that the patterns of action potential waveforms are somewhat similar in rodents (rat or mouse), although the APD is shorter in mouse, and the complement of the Kþ currents underlying the cardiac repolarization in mouse are also different than those in rat (45,46). The cardiac repolarization in rat is controlled by two distinct depolarization activated Kþ currents, the Ca2þ-independent transient outward Kþ current (It) and the steady-state outward Kþ current (Iss), (40,47). In mouse ventricular myocytes, an additional current, the 4-AP sensitive (at concentrations less than 100 mM), slowly
inactivating, delayed rectifier Kþ current (IKslow) has been deemed to play an important role (41,48). The properties
of the depolarization-activated Kþ currents have now been well characterized in rats (40,49) and mouse (43,48), and appear to be significantly different. It is therefore interesting to investigate in computational modeling whether the reported differences in the properties of the depolarization-activated Kþ currents can account for the dissimilar nature of the action potential configurations observed in rats and mice.
COMPUTATIONAL MODELING OF THE MURINE VENTRICULAR ACTION POTENTIALS
The goal of my computational modeling laboratory has been to unify different experimental data and to develop biophysically detailed models for the rat and mouse ventricular cells and to determine the underlying ionic channels responsible for differences in cardiac action potential variations in rats and mice under normal and diseased conditions. A computational model has been developed for the rat cardiac ventricular cell based on electrophysiology data. Our control model (12) represents the bioelectric activity in the left ventricular cells in adult male rats. The differences in the membrane properties within the left ventricle to simulate the action potential variations of the endocardial and epicardial cells have been formulated. Also, a right ventricular cell model from our control model was built (the left ventricular cell model) to investigate ionic mechanisms in diabetic rats (33). Our right ventricular cell model was also the template for us to develop a mouse ventricular cell model by utilizing experimental data (8,32).
The left (LV) and right (RV) ventricular cell models for the rat consist of a Hodgkin–Huxley type membrane model that is described by the membrane capacitance, various ionic channels; the fast Naþ current (INa), long-lasting
Ca2þ current (ICaL), the 4AP sensitive, Ca2þ independent transient outward Kþ current (It), steady-state outward Kþ
current (Iss), inward rectifier Kþ current (IK1), hyperpolarization activated current (If), linear background current
(IB); the Naþ/Ca2þ ion exchanger (INaCa), and the Naþ/Kþ (INaK) and Ca2þ membrane (ICaP) pumps, that are experimentally observed in rat ventricular cells.
The mouse ventricular cell model was constructed by using the rat right ventricular cell model as the template. The mouse LV apex cell was developed by adding the 4AP sensitive slowly inactivating, delayed rectifier Kþ current
(IKslow) based on the data of Fiset et al. (41) and Zhou et al. (48), and by reformulating It and Iss based on experiments
performed by Agus et al. (35–37) and Xu et al. (43) in mice. Further, a mouse LV septum cell model was developed by formulating a new current Itos based on the data of (Xu et
al. (43)), and by reducing the densities of Itof, IKslow, and Iss by 70, 23, and 30%, respectively, based on data of Gussak
et al. (45).
The important results of our simulation studies are
1.The action potential heterogeneity (Fig. 3) in the adult rat LV is mainly due to the changes in the den-
sity and recovery kinetics of It and due to the altered density of INa (12).
2.The RV cell model can be developed from the LV cell
model by changing the densities of It, Iss, ICaL, and INaK based on experimental data.
3.The changes in the density and the reactivation
kinetics of It can account for the action potential prolongation differences in RV myocytes of diabetic (type-I, short term) rats (33) and LV myocytes of spontaneously hypertensive rats (35) (Fig. 4).
4.The presence of IKslow in mouse is one of the main factors contributing to the faster rate of repolarization seen in mouse compared to rats (Fig. 5) (8).
5.The LV septum cell model had more prolonged action potentials than the apex cells and these simulation results (Fig. 6a) are qualitatively similar to the experimental data of (52) (Fig. 6b).
Simulated Action Potentials of the Epicardial and Endocardial Cardiac Ventricular Cells
60
(mV)Voltage |
0 |
|
|
|
|
|
|
|
EPI |
ENDO |
|
|
–80 |
|
|
|
0 |
100 |
200 |
|
|
Time (ms) |
|
Figure 3. Simulated action potentials of the rat left ventricular (LV) epicardial (EPI) (solid line) and endocardial (ENDO) (dashed line) cells (35–37).
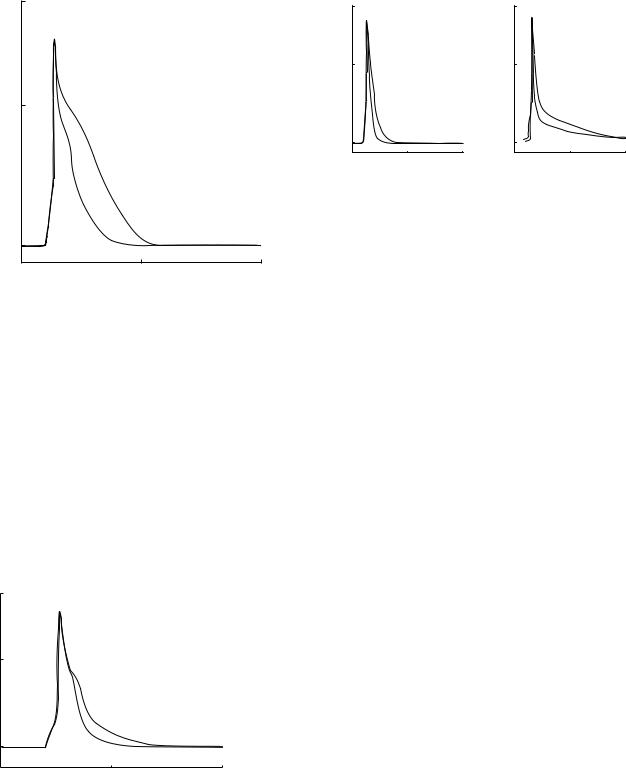
Simulated Action Potentials of the Epicardial Cardiac Ventricular Cells for Normal and Spontaneously Hypertensive Rats
60
(mV)Voltage |
0 |
|
SH
N
–80
0 |
100 |
200 |
Time (ms)
Figure 4. Model generated ventricular action potentials of the epicardial cells for the normal rat (N) (solid line) and spontaneously hypertensive (SH) rat (dashed line) (35–37).
6.The rat epicardial and endocardial ventricular cell models were more rate-sensitive than the mouse ventricular cell model and these simulation data match the experimental data well.
In conclusion, the mathematical modeling study of murine ventricular myocytes complements our knowledge of the biophysical data with simulation data and provide us with quantitative descriptions to understand the ionic currents underlying the cardiac action potential variations in different species. This kind of computational work will
Simulated Cardiac Action Potentials of Rat and Mouse Ventricular Cells
|
60 |
|
|
|
(mV)Voltage |
0 |
|
|
|
|
|
|
|
|
|
|
M |
R |
|
|
|
|
|
|
|
–80 |
|
|
|
|
0 |
|
50 |
100 |
|
|
|
Time (ms) |
|
Figure 5. Simulated action potentials of the mouse left ventricular apex cell (M) (solid line) and the rat right ventricular cell (R) (dashed line) (35–37).
ELECTROPHYSIOLOGY 147
Simulated Ventricular Action Potentials for
Mouse LV Apex and Septum Cells
|
60 |
Model |
|
|
60 |
Experiments |
|
|
|
|
|
|
|
||
(mV) |
0 |
|
|
(mV) |
0 |
|
|
Voltage |
|
|
Voltage |
|
|
||
|
|
|
|
S |
|
||
|
|
S |
|
|
|
|
|
|
|
|
|
|
A |
|
|
|
–80 |
A |
|
|
–80 |
|
|
|
|
|
|
|
|||
|
0 |
100 |
200 |
|
0 |
100 |
200 |
|
|
Time (ms) |
|
|
|
Time (ms) |
|
|
|
(a) |
|
|
|
(b) |
|
Figure 6. Simulated and experimentally recorded action potentials of the mouse left ventricular apex (A) (solid line) and the septum (S) (dashed line) cells (35–37).
enhance our understanding of the ionic mechanisms that contribute to the cardiac action potential variation in normal and diseased animals, and will provide us with better treatments for diseases in humans.
DISSEMINATION OF COMPUTATIONAL MODELS
My computational modeling laboratory has developed an interactive cell modeling web site, iCell (http://ssd1.bme. memphis.edu/icell/) since 1998. iCell, that integrates research and education, was specifically developed as a simulation-based teaching and learning resource for electrophysiology (35–37,51,52). The main objectives for the development of iCell were (1) to use technology in electrophysiology education, (2) to provide a learning and teaching resource via internet for cellular electrophysiology, (3) to allow the user to understand the cellular physiology mechanisms, membrane transport and variations of action potentials and ion channels by running simulations, and (4) to provide a computer platform independent resource for cellular models to be used for teaching, learning and collaboration. The site consists of JAVA applets representing models of various cardiac cells and neurons, and provides simulation data of their bioelectric transport activities at cellular level. Each JAVA-based model allows the user to go through menu options to change model parameters, run and view simulation results. The site also has a glossary section for the scientific terms. iCell has been used as a teaching and learning tool for seven graduate courses at the Joint Biomedical Engineering Program of University of Memphis and University of Tennessee. This modeling tool was also used as a collaboration site among our colleagues interested in simulations of cell membrane activities. Scientists from the fields of biosciences, engineering, life sciences and medical sciences in 17 countries, Argentina, Belgium, Brazil, Canada, China, England, Germany, Greece, Ireland, Japan, Korea, the Netherlands, New Zealand, Spain, Taiwan, Turkey and the United States, have tested and utilized iCell as a
148 ELECTROPHYSIOLOGY
simulation-based teaching, learning and collaboration environment. The platform-independent software, iCell, provides us with an interactive and user-friendly teaching and learning resource, and also a collaboration environment for electrophysiology to be shared over the Internet. The usage of simulations for teaching and learning will continue advancing simulation-based engineering and sciences for research and development.
The simulations provided by iCell and other resources, such as CellML (http://www.cellml.org/public/news/ index.html), Virtual Cell (http://www.nrcam.uchc.edu/), JSIM (http://nsr.bioeng.washington.edu/PLN/Software/), simBio Cell/Biodynamics Simulation Project of Kyoto University (http://www.biosim.med.kyoto-u.ac.jp/e/index. html), and E-Cell (http://ecell.sourceforge.net/), will continue signifying the important verification and prediction capabilities of the computer models to represent, analyze and complement the physiological data and knowledge. The model development demonstrates that computational models have to be constructed from experimental electrophysiology data, not only to explain and to verify the data that they are based on, but also to predict results for experiments that have not been performed and to guide future experiments.
Overall, computational modeling and simulation results continue to advance our understanding of living systems at the cellular level in cardiac electrophysiology while promoting collaborations and training in interdisciplinary field of bioengineering between scientists in life scientists and engineers. The presentation of computational models in user friendly, interactive and menu driven software are important in bringing collaborators of different disciplines and training scientists and students in cross-disciplinary projects.
IMPACT OF COMPUTATIONAL MODELING IN VENTRICULAR CELLS
The following summarizes impacts of the computational model development of ventricular bioelectric activity and the model-generated data in different disciplines of life sciences. I. Biophysics and Physiology: The results of the computational studies expand our knowledge of the living systems at the cellular level in electrophysiology. II. Clinical Physiology and Medicine: The insights gained and conclusions derived from the computational studies enhance our understanding of the biocomplexity of the heart, and provide us with better knowledge to be used in the future in treatments for diseases in humans. The cardiac cells’ responses to various pathophysiological states with simulation data will also be better understood. III. Pharmacology: The differences in ventricular membrane ionic currents, especially outward Kþ currents in different species have very important practical implications. Different drugs are known to affect different ionic currents and to change action potential waveforms in different mammalian heart preparations under various conditions of development, aging and gender. A better understanding of the role of the ionic currents that control repolarization in the ventricular myocytes obtained from various species including rat and mouse, as presented
in this paper, will provide motivation and explanations for species differences in treatment and drug actions, and also promote pharmacological research that may lead to the development of more specific drugs to be used in children and adults.
ACKNOWLEDGMENTS
These computational research projects presented here were funded by the Whitaker Foundation (PI: Dr. S. S. Demir). The author acknowledges the contributions of her former students S. Pandit, S. Padmala, and E. Damaraju to these research projects.
The author thanks her former students, Joe E. McManis, Yiming Liu, Dong Zhang, Srikanth Padmala and Eswar Damaraju for coding JAVA applets in the iCell project, her students Chris Oehmen and Jing Zheng for posting the html pages and Dr. Emre Velipasaoglu, Siddika Demir and Asim Demir for valuable collaborations and discussions. This research was also funded by the Whitaker Foundation (PI, Dr. S. S. Demir).
BIBLIOGRAPHY
Cited References
1.Alberts B, et al. Membrane Transport. Essential Cell Biology: An Introduction to the Molecular Biology of the Cell. New York: Garland Publishing; 1997 p 371–407. Chapt. 12
2.Plonsey R, Barr R. Bioelectricity: A Quantitaive Approach. New York: Kluwer Academic Publications; 2000.
3.Hamill O, et al. Improved patch-clamp techniques for highresolution current recording from cells and cell-free membrane patches. Pflugers Archiv 1981;391:85–100.
4.Neher E, Sakmann B. Noise analysis of drug induced voltage clamp currents in denervated frog muscle fibres. J Physiol 1976;258(3):705–729.
5.Neher E, Sakmann B. Single-channel currents recorded from membrane of denervated frog muscle fibres. Nature (London) 1976;260(5554):799–802.
6.Neher E, Sakmann B, Steinbach JH. The extracellular patch clamp: a method for resolving currents through individual open channels in biological membranes. Pflugers Arch 1978;375(2):219–228..
7.Neher E, Sakmann B. The patch clamp technique. Sci Am 1992;266(3):44–51.
8.Damaraju E. A Computational Model of Action Potential Heterogeneity in Adult Mouse Left Ventricular Myocytes. M.S. dissertation, University of Memphis, 2003.
9.Fozzard H. Cardiac Electrogenesis and the Sodium Channel. In: Spooner P, Brown A, Catterall W, Kaczorowski G, Strauss H, editors. Ion Channels in the Cardiovascular system:Function and dysfunction. Futura Publishing Company, Inc.; 1994. Chapt. 5.
10.Spooner PM, Rosen MR, editors. Foundations of Cardiac Arrhythmias. 1st ed. New York: Marcel Dekker; 2000.
11.Demir SS, et al. Action Potential Variation in Canine Ventricle: A Modeling Study. Comput Cardiol 1996; 221–224.
12.Pandit SV, Clark RB, Giles WR, Demir SS. A Mathematical Model of Action Potential Heterogeneity in Adult Rat Left Ventricular Myocytes. Biophy J 2001;81:3029–3051.
13.Bers DM. Excitation-Contraction Coupling and Cardiac Contractile Force 2nd ed. The Netherlands: Kluwer Academic Publications; 2001.
14.Hodgkin L, Huxley AF. A quantitative description of membrane current and its application to conduction and excitation in nerve. J Physiol 1952;117:500–544.
15.Irvine L, Jafri M, Winslow R. Cardiac sodium channel Markov model with temperature dependence and recovery from inactivation. Biophysic J 1999;76(4):1868–1885.
16.Beeler GW, Reuter H. Reconstruction of the action potential of ventricular myocardial fibres. J Physiol 1997;268: 177–210.
17.Drouhard J, Roberge FA, Revised formulation of the Hodg- kin-Huxley representation of the sodium current in cardiac cells. Comput Biomed Res 1987;20:333–350.
18.Winslow RL, et al. Mechanisms of altered excitation-contrac- tion coupling in canine tachycardia-induced heart failure, II: model studies. Circ Res 1999;84:571–586.
19.Fox JJ, McHarg JL, Gilmour RF. Ionic mechanism of electrical alternans. Am J Physiol Heart Circ Physiol 2002;282:H516–H530.
20.Nordin C, Computer model of membrane current and intracellular Ca2þ flux in the isolated guinea pig ventricular myocyte. Amer J Physiolo 1993;265:H2117–H2136.
21.Luo C-H, Rudy Y. A model of the ventricular cardiac action potential. Circulation Res 1991;68:1501–1526.
22.Luo C-H, Rudy Y. A dynamic model of the cardiac ventricular action potential. I Simluation of ionic currents and concentration changes. Circulation Res 1994;74:1071–1096.
23.Zeng J, Laurita K, Rosenbaum DS, Rudy Y. Two components of the delayed rectifier Kþ current in ventricular myocytes of the guinea pig type: theoretical formulation and their role in repolarization. Circulation Res 1995;77:140–152.
24.Noble D, Varghese A, Kohl P, Noble P. Improved guinea-pig ventricular cell model incorporating a diadic space, IKr and IKs, and lengthand tension-dependent processess. Can J Cardiol 1998;14:123–134.
25.Priebe L, Beuckelmann D. Simulation study of cellular electric properties in heart failure. Circ Res 1998;82: 1206– 1223.
26.ten Tusscher KHWJ, Noble D, Noble PJ, Panfilov AV. A model for human ventricular tissue.Am J Physiol Heart Circ Physiol 2004;286:H1573–H1589.
27.Riemer TL, Sobie A, Tung L. Stretch-induced changes in arrhythmogenesis and excitability in experimentally based heart cell models. Am J Physiol 1998;275:H431–H442.
28.Puglisi JL, Bers DM. LabHEART: an interactive computer model of rabbit ventricular myocyte ion channels and Ca transport. Am J Physiol Cell Physiol 2001;281:C2049–C2060.
29.Winslow RL, et al. Electrophysiological modeling of cardiac ventricular function: from cell to organ. Annu Rev Biomed Eng 2000;2:119–155.
30.Members of the Sicilian Gambit. New approaches to antiarrhythmic therapy, Part I: Emerging therapeutic applications of the cell biology of cardiac arrhythmias. Circulation 2001;104:2865–2873.
31.Bouchard RA, Clark RB, Giles WR. Effects of action potential duration on excitation-contraction coupling in rat ventricular myocytes. Action potential voltage-clamp measurements. Circ Res 1995;76:790–801.
32.Pandit SV. Electrical Activity in Murine Ventricular Myocytes: Simulation Studies. Ph.D. dissertation, University of Memphis; 2002.
33.Pandit SV, Giles WR, Demir SS. A Mathematical Model of the Electrophysiological Alterations in Rat Ventricular Myocytes in Type-I Diabetes. Biophys J 2003;84(2):832–841.
34.Padmala S, Demir SS. A computational model of the ventricular action potential in adult spontaneously hypertensive rats. J Cardiovasc Electrophysiol 2003;14:990– 995.
ELECTROPHYSIOLOGY 149
35.Demir SS. Computational Modeling of Cardiac Ventricular Action Potentials in Rat and Mouse. Rev Jne J Physiol 2004;54(6):523–530.
36.Demir SS. An Interactive Electrophysiology Training Resource for Simulation-Based Teaching and Learning. Institute of Electrical and Electronics Engineers (IEEE) Engineering in Medicine & Biology Society Proceedings; 2004. p 5169–5171.
37.Demir SS. The Significance of Computational Modelling in Murine Cardiac Ventricular Cells. J Appl Bionics Biomech 2004;1(2):107–114.
38.Antzelevitch C, Yan G-X, Shimuzu W, Burashnikov A. Electrical Heterogeneity, the ECG, and Cardiac Arrhythmias. In: Zipes DP, Jalife J, editors. Cardiac Electrophysiology: From Cell to Bedside. 3rd ed. Philadelphia: WB Saunders; 1999. p 222–238.
39.Watanabe T, Delbridge LM, Bustamante JO, McDonald TF. Heterogeneity of the action potential in isolated rat ventricular myocytes and tissue. Circ Res 1983;52:280–290.
40.Clark RB, et al. Heterogeneity of action potential waveforms and potassium currents in rat ventricle. Cardiovas Res 1993;27:1795–1799.
41.Fiset C, Clark RB, Larsen TS, Giles WR. A rapidly activating sustained Kþ current modulates repolarization and excita- tion-contraction coupling in adult mouse ventricle. J Physiol 1997;504:557–563.
42.Nerbonne JM, Nichols CG, Schwarz TL, Escande D. Genetic manipulation of cardiac Kþ channel function in mice: what have we learned, and where do we go from here? Circ Res 2001;89:944–956.
43.Xu H, Guo W, Nerbonne JM. Four kinetically distinct depo- larization-activated Kþ currents in adult mouse ventricular myocytes. J Gen Physiol 1999;113:661–678.
44.Chien KR. To Cre or not to Cre: the next generation of mouse models of human cardiac diseases. Circ Res 2001;88:546– 549.
45.Gussak I, Chaitman BR, Kopecky SL, Nerbonne JM. Rapid ventricular repolarization in rodents: electrocardiographic manifestations, molecular mechanisms, and clinical insights. J Electrocardiol 2000;33:159–170.
46.Nerbonne JM. Molecular analysis of voltage-gated Kþ channel diversity and functioning in the mammalian heart. In: Page E, Fozzard HA, Solaro RJ, editors. Handbook of Physiology: The Cardiovascular System. New York: Oxford University Press; 2001. 568–594.
47.Shimoni Y, Severson D, Giles WR. Thyroid status and diabetes modulate regional differences in potassium currents in rat ventricle. J Physiol 1995;488:673–688.
48.Zhou J, et al. Characterization of a slowly inactivating outward current in adult mouse ventricular myocytes. Circ Res 1998;83:806–814.
49.Shimoni Y, Light PE, French RJ. Altered ATP sensitivity of ATP-dependent Kþ channels in diabetic rat hearts. Am J Physiol 1998;275:E568–E576.
50.Demir SS. iCell: an Interactive Web Resource for SimulationBased Teaching and Learning in Electrophysiology Training. Institute of Electrical and Electronics Engineers (IEEE), Engineering in Medicine & Biology Society Proceedings; 2003; p 3501–3504.
51.Demir SS. Simulation-based Training in Electrophysiology by iCell Institute of Electrical and Electronics Engineers, Engineering in Medicine and Biology Engineering in Medicine & Biology Society Proceedings, 4 pages; 2005.
See also BLADDER DYSFUNCTION, NEUROSTIMULATION OF; ELECTROCONVULSIVE THERAPY; PACEMAKERS; SPINAL CORD STIMULATION; TRANSCUTANEOUS ELECTRICAL NERVE STIMULATION (TENS).