
- •VOLUME 4
- •CONTRIBUTOR LIST
- •PREFACE
- •LIST OF ARTICLES
- •ABBREVIATIONS AND ACRONYMS
- •CONVERSION FACTORS AND UNIT SYMBOLS
- •HYDROCEPHALUS, TOOLS FOR DIAGNOSIS AND TREATMENT OF
- •HYPERALIMENTATION.
- •HYPERBARIC MEDICINE
- •HYPERBARIC OXYGENATION
- •HYPERTENSION.
- •HYPERTHERMIA, INTERSTITIAL
- •HYPERTHERMIA, SYSTEMIC
- •HYPERTHERMIA, ULTRASONIC
- •HYPOTHERMIA.
- •IABP.
- •IMAGE INTENSIFIERS AND FLUOROSCOPY
- •IMAGING, CELLULAR.
- •IMAGING DEVICES
- •IMMUNOLOGICALLY SENSITIVE FIELD–EFFECT TRANSISTORS
- •IMMUNOTHERAPY
- •IMPEDANCE PLETHYSMOGRAPHY
- •IMPEDANCE SPECTROSCOPY
- •IMPLANT, COCHLEAR.
- •INCUBATORS, INFANTS
- •INFANT INCUBATORS.
- •INFUSION PUMPS.
- •INTEGRATED CIRCUIT TEMPERATURE SENSOR
- •INTERFERONS.
- •INTERSTITIAL HYPERTHERMIA.
- •INTRAAORTIC BALLOON PUMP
- •INTRACRANIAL PRESSURE MONITORING.
- •INTRAOCULAR LENSES.
- •INTRAOPERATIVE RADIOTHERAPY.
- •INTRAUTERINE DEVICES (IUDS).
- •INTRAUTERINE SURGICAL TECHNIQUES
- •ION-EXCHANGE CHROMATOGRAPHY.
- •IONIZING RADIATION, BIOLOGICAL EFFECTS OF
- •ION-PAIR CHROMATOGRAPHY.
- •ION–SENSITIVE FIELD-EFFECT TRANSISTORS
- •ISFET.
- •JOINTS, BIOMECHANICS OF
- •JOINT REPLACEMENT.
- •LAPARASCOPIC SURGERY.
- •LARYNGEAL PROSTHETIC DEVICES
- •LASER SURGERY.
- •LASERS, IN MEDICINE.
- •LENSES, CONTACT.
- •LENSES, INTRAOCULAR
- •LIFE SUPPORT.
- •LIGAMENT AND TENDON, PROPERTIES OF
- •LINEAR VARIABLE DIFFERENTIAL TRANSFORMERS
- •LITERATURE, MEDICAL PHYSICS.
- •LITHOTRIPSY
- •LIVER TRANSPLANTATION
- •LONG BONE FRACTURE.
- •LUNG MECHANICS.
- •LUNG PHYSIOLOGY.
- •LUNG SOUNDS
- •LVDT.
- •MAGNETIC RESONANCE IMAGING
- •MAGNETOCARDIOGRAPHY.
- •MANOMETRY, ANORECTAL.
- •MANOMETRY, ESOPHAGEAL.
- •MAMMOGRAPHY
- •MATERIALS, BIOCOMPATIBILITY OF.
- •MATERIALS, PHANTOM, IN RADIOLOGY.
- •MATERIALS, POLYMERIC.
- •MATERIALS, POROUS.
- •MEDICAL EDUCATION, COMPUTERS IN
- •MEDICAL ENGINEERING SOCIETIES AND ORGANIZATIONS
- •MEDICAL GAS ANALYZERS
- •MEDICAL PHOTOGRAPHY.
- •MEDICAL PHYSICS LITERATURE
- •MEDICAL RECORDS, COMPUTERS IN
- •MICROARRAYS
- •MICROBIAL DETECTION SYSTEMS
- •MICROBIOREACTORS
- •MICRODIALYSIS SAMPLING
- •MICROFLUIDICS
- •MICROPOWER FOR MEDICAL APPLICATIONS
- •MICROSCOPY AND SPECTROSCOPY, NEAR-FIELD
- •MICROSCOPY, CONFOCAL
- •MICROSCOPY, ELECTRON
- •MICROSCOPY, FLUORESCENCE
- •MICROSCOPY, SCANNING FORCE
- •MICROSCOPY, SCANNING TUNNELING
- •MICROSURGERY
- •MINIMALLY INVASIVE SURGICAL TECHNOLOGY
- •MOBILITY AIDS
- •MODELS, KINETIC.
- •MONITORING IN ANESTHESIA
- •MONITORING, AMBULATORY.
- •MONITORING, FETAL.
- •MONITORING, HEMODYNAMIC
- •MONITORING, INTRACRANIAL PRESSURE
- •MONITORING, NEONATAL.
- •MONITORING, UMBILICAL ARTERY AND VEIN
- •MONOCLONAL ANTIBODIES
- •MOSFET.
- •MUSCLE ELECTRICAL ACTIVITY.
- •MUSCLE TESTING, REHABILITATION AND.
- •MUSCULOSKELETAL DISABILITIES.
516MICROSCOPY, SCANNING TUNNELING
39.Hutter JL, Bechhoefer J. Calibration of atomic-force
microscope tips. Rev Sci Instrum 1993;64(7):1868– 1873.
40.Bell GI. Models for the specific adhesion of cells to cells. Science 1978;200:618–627.
41.Merkel R, et al. Energy landscapes of receptor-ligand bonds explored with dynamic force spectroscopy [see comments]. Nature 1999;397(6714):50–53.
42.Tees DFJ, Woodward JT, Hammer DA. Reliability theory for receptorligand bond dissociation. J Chem Phys 2001;114: 7483–7496.
43.Evans E. Probing the relation between force—lifetime—and chemistry in single molecular bonds. Ann Rev Biophys Biomolec Struc 2001;30:105–128.
44.Hoh JH, Schoenenberger CA. Surface morphology and mechanical properties of MDCK monolayers by atomic force microscopy. J Cell Sci 1994;107:1105–1114.
45.Radmacher M, et al. Measuring the viscoelastic properties of human platelets with the atomic force microscope. Biophys J 1996;70(1):556–567.
46.Wu HW, Kuhn T, Moy VT. Mechanical properties of L929 cells measured by atomic force microscopy: Effects of anticytoskeletal drugs and membrane crosslinking. Scanning 1998;20(5): 389–397.
47.Fisher TE, et al. The study of protein mechanics with the atomic force microscope. Trends Biochem Sci 1999;24(10):379–384.
48.Altmann SM, Lenne P-F. Forced unfolding of single proteins. Methods Cell Biol 2002;68:312–336.
49.Hafner JH, et al. Structural and functional imaging with carbon nanotube AFM probes. Prog Biophys Molec Biol 2001;77(1):73–110.
50.Yang Y, Wang H, Erie DA. Quantitative characterization of biomolecular assemblies and interactions using atomic force microscopy. Methods 2003;29(2):175–187.
51.Sheng S, Zhifeng S. Cryo-atomic force microscopy. Methods Cell Biol 2002;68:243–256.
52.Fotiadis D, et al. Imaging and manipulation of biological structures with the AFM. Micron 2002;33(4):385–397.
53.Winfree E, et al. Design and self-assembly of two-dimensional DNA crystals. Nature 1998;394(6693):539–544.
54.Hansma HG, Kasuya K, Oroudjev E. Atomic force microscopy imaging and pulling of nucleic acids. Curr Opin Struct Biol 2004;14(3):380–385.
55.Jaeger L, Westhof E, Leontis NB. TectoRNA: Modular assembly units for the construction of RNA nano-objects. Nucl Acids Res 2001;29:455–463.
56.Seeman NC. DNA in a material world. Nature 2003;421:427– 431.
57.Yan H, et al. DNA-templated self-assembly of protein arrays and highly conductive nanowires. Science 2003;301(5641): 1882–1884.
58.Seeman NC. From genes to machines: DNA nanomechanical devices. Trends Biochem Sci 2005;30(3):119–125.
59.Radmacher M. Measuring the elastic properties of living cells by AFM. Methods Cell Biol 2002;68:67–90.
60.Langer MG, Koitschev A. The biophysics of sensory cells of the inner ear examined by AFM and patch clamp. Methods Cell Biol 2002;68:142–171.
61.Charras GT, Lehenkari P, Horton M. Biotechnological applications of AFM. Methods Cell Biol 2002; 68.
62.Charras GT, Horton MA. Single cell mechanotransduction and its modulation analyzed by atomic force microscope indentation. Biophys J 2002;82(6):2970–2981.
See also BIOMAGNETISM; NANOPARTICLES.
MICROSCOPY, SCANNING TUNNELING
VIRGINIA M. AYRES
Michigan State University
East Lansing, Michigan
INTRODUCTION
Four years after its invention in 1982 (1), the scanning tunneling microscope (STM) was awarded the 1986 Nobel Prize for physics, one of only four such prestigious awards given for a truly significant contribution to scientific instrumentation. Since then, the family of scanning probe microscopy (SPM) techniques, which includes scanning tunneling microscopy, atomic force microscopy (2–4), magnetic force microscopy (5), near-field optical microscopy (6), scanning thermal microscopy (7), and others, has revolutionized studies of semiconductors, polymers, and biological systems. The key capability of SPM is that, through a controlled combination of feedback loops and detectors with the raster motion of piezoelectric actuator, it enables direct investigations of atomic-to-nanometer scale phenomena.
Scanning probe microscopy is based on a piezoelectricactuated relative motion of a tip versus sample surface, while both are held in a near-field relationship with each other. In standard SPM imaging, some type of tip-sample interaction (e.g., tunneling current, Coulombic forces, magnetic field strength) is held constant in z through the use of feedback loops, while the tip relative to the sample undergoes an x–y raster motion, thereby creating a surface map of the interaction. The scan rate of the x–y raster motion per line is on the order of seconds while the tip-sample interaction is on the order of nanoseconds or less. The SPM is inherently cable of producing surface maps with atomic scale resolution, although convolution of tip and sample artifacts must be considered.
Scanning tunneling microscopy is based on a tunneling current from filled to empty electronic states. The selectivity induced by conservation of energy and momentum requirements results in a self-selective interaction that gives STM the highest resolution of all scanning probe techniques. Even with artifacts, STM routinely produces atomic scale (angstrom) resolution.
With such resolution possible, it would be highly desirable to apply STM to investigations of molecular biology and medicine. Key issues in biology and medicine revolve around regulatory signaling cascades that are triggered through the interaction of specific macromolecules with specific surface sites. These are well within the inherent resolution range of STM.
The difficulty when considering the application of STM to molecular biology is that biological samples are nonconductive. It may be more accurate to describe biological samples as having both local and varying conductivities. These two issues will addressed in this article, and examples of conditions for the successful use of STM for biomedical imaging will be discussed. We begin with an overview of successful applications of STM in biology and medicine.
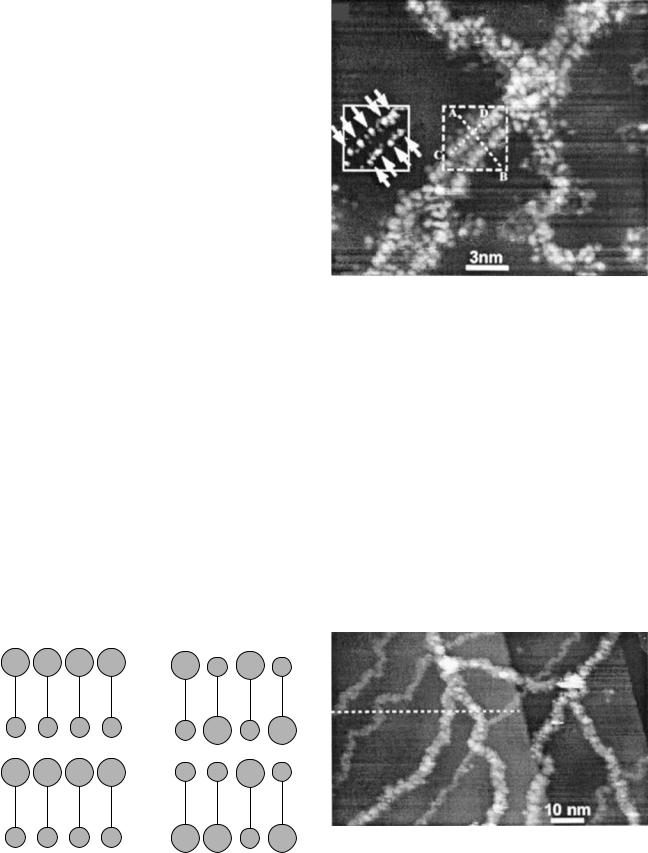
SCANNING TUNNELING MICROSCOPY IN BIOLOGY AND MEDICINE: DNA AND RNA
The STM imaging for direct analysis of base pair arrangements in DNA was historically the first biological application of the new technique. An amusing piece of scientific history is that the first (and widely publicized) images (8– 12) of (deoxyribonucleic acid) DNA were subsequently shown to correspond to electronic sites on the underlying graphite substrate! However, more careful investigations have resulted in an authentic body of work in which the base pairings and conformations of DNA and RNA are directly investigated by STM. One goal of these investigations is to replace bulk sequencing techniques and crystal diffraction techniques, which both require large amounts of material, with the direct sequencing of single molecules of DNA and RNA. Two examples of DNA and RNA investigation by STM are presented here. One is an investigation of DNA and RNA structures, and the other is an investigation of DNA biomedical function.
Recently reported research from the group at The Institute for Scientific and Industrial Research at Osaka University in Japan (13) has shown detailed STM images of well-defined guanine-cytosine (G-C) and adenine-thymine (A-T) base pairings in doubleand single-stranded DNA. Four simple samples involving only G-C and only A-T base pairs in mixed (hetero) and single sided (homo) combinations were chosen for analysis (Fig. 1). These were deposited on a single-crystal copper (111)-orientation [Cu(111)] substrate using a technique developed specially by this group to produce flat, extended strands for imaging. An STM image showing the individual A-T base pairs in the hetero A-T sample is shown in Fig. 2. Images of the overall structures indicated repeat distances consistent with interpretation as the double helix. Images from mixed samples of hetero G-C and hetero A-T are shown in Fig. 3. The larger structure is interpreted as hetero G-C and the smaller as hetero A-T, which is consistent with X-ray diffraction data that indicates the A-T combination is more compact.
Only the double helix structure was observed for the hetero G-C samples. However, the homo G-C structures,
(a) |
|
|
|
(b) |
|
|
|
|
A |
A |
A |
A |
|
A |
T |
A |
T |
T |
T |
T |
T |
|
T |
A |
T |
A |
(c) G |
G |
G |
G |
(d) |
C |
C |
G |
C |
MICROSCOPY, SCANNING TUNNELING |
517 |
Figure 2. STM image of portion of Hetero A-T double helix of showing base pairs. (Reproduced from Ref. 9, used with permission.)
hetero A-T structures, and homo A-T structures were observed in two types, and the spot spacings and sizes of the second type would be consistent with interpretation as single-stranded DNA. The observed presence or lack of single-stranded configurations among the samples is consistent with the fact that hetero G-C has a higher melting (unraveling) temperature than the homo G-C and thus is more difficult to unwind. Both hetero and homo A-T pairs have lower melting temperatures than either of the G-C pairs. Images of both hetero A-T and Homo A-T samples often showed sizing and spacings consistent with interpretation as single-stranded DNA, in addition to observed double helix specimens. Thus, the presence/lack of singlestranded versus double helix images is consistent with known melting temperature data for the C-G and A-T base pairings.
The same group has also reported successful STM investigations of transfer-ribonuclic acid (t-RNA) (14). In
C C C C G G C G
Figure 1. (a) Homo A-T, (b) Hetero A-T, (c) Homo G-C, and (d) Hetero G-C. (Figure adapted from Ref. 9, used with permission.)
Figure 3. Hetero G-C and Hetero A-T mixed sample. The larger specimens are identified as Hetero G-C, and the smaller specimens are identified as Hetero A-T. Both are in a double helix configuration. (Reproduced from Ref. 9, used with permission.)
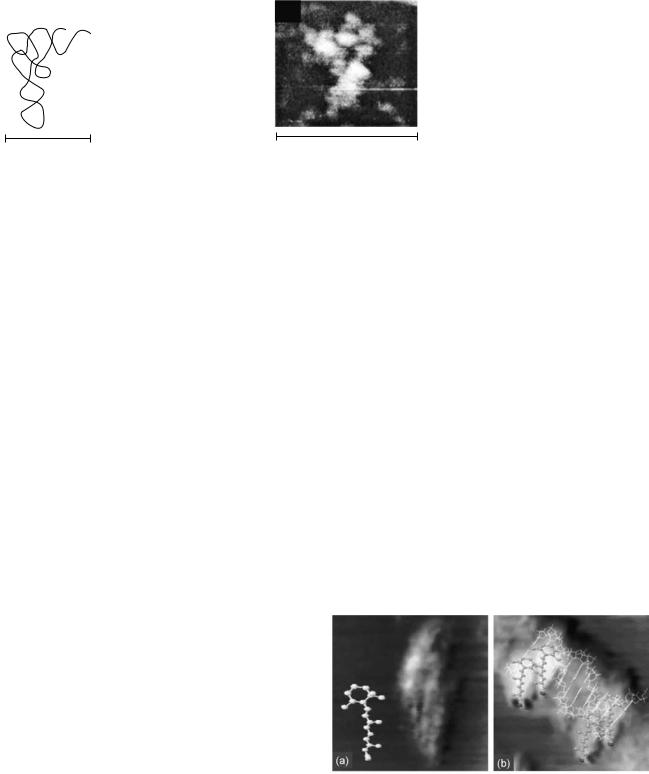
518 MICROSCOPY, SCANNING TUNNELING
(a) |
(b) |
accepter stem
TΨC arm
D arm
anticodon arm
6 nm
|
|
|
|
|
|
|
|
|
|
|
|
|
|
|
|
|
3′ |
|
|
|
|
|
|
|
|
|
|
|
|
|
|
|
|
|
|
|
|
|
|
|
A |
|
|
|
|
|
|
|
|
|
|
|
|
|
|
|
|
|
|
|
|
|
|
|
C |
|
|
|
|
|
|
|
|
|
|
|
|
|
|
|
|
|
|
5′ |
C |
accepter stem |
|||||||||
|
|
|
|
|
|
|
|
|
|
|
|
G |
|
CA |
|||||||||
|
|
|
|
|
|
|
|
|
|
|
|||||||||||||
|
|
|
|
|
|
|
|
|
|
|
|
G |
|
C |
|
|
|
|
|
||||
|
|
|
|
|
|
|
|
|
|
|
|
|
|
|
|
||||||||
|
|
|
|
|
|
|
|
|
|
|
|
G |
|
C |
|
|
|
|
|
||||
|
|
|
|
|
|
|
|
|
|
|
|
|
|
|
|
||||||||
|
|
|
|
|
|
|
|
|
|
|
|
U |
|
A |
|
|
|
|
|
||||
|
|
|
|
|
|
|
|
|
|
|
|
|
|
|
|
||||||||
|
|
|
|
|
|
|
|
|
|
|
|
C |
|
G |
TΨC arm |
||||||||
|
|
|
|
|
|
|
|
|
|
|
|||||||||||||
D arm |
|
|
|
G |
|
C |
|||||||||||||||||
|
|
||||||||||||||||||||||
|
|
|
U |
|
A |
|
|
|
|
U A A |
|||||||||||||
|
|
|
|
|
|
||||||||||||||||||
G |
|
U |
CGUCC |
G |
|||||||||||||||||||
A |
|
|
|
|
|
|
|||||||||||||||||
UU A |
|
CUCG |
CGCAGG UUC |
||||||||||||||||||||
G |
|
|
|
|
|
|
|
|
|
|
|
|
|
|
|
|
U |
|
|
|
|
|
|
|
|
|
|
|
|
|
|
|
|
|
|
|
|
|
|
|
|||||||
G U AGAGCA |
|
|
|
|
|
|
U G G |
|
|||||||||||||||
|
|
|
|
|
|
|
|
|
G |
|
U |
|
|
extra loop |
|||||||||
|
|
|
|
|
|
|
|
|
|
|
|
||||||||||||
|
|
|
|
|
|
|
|
|
U |
|
A |
|
|
|
|
|
|
|
|||||
|
|
|
|
|
|
|
|
|
|
|
|
|
|
|
|
|
|||||||
|
|
|
|
|
|
|
|
|
U |
|
A |
|
|
|
|
|
|
|
|||||
|
|
|
|
|
|
|
|
|
|
|
|
|
|
|
|
|
|||||||
|
|
|
|
|
|
|
|
|
G |
|
C |
anticodon arm |
|||||||||||
|
|
|
|
|
|
|
|
|
|
||||||||||||||
|
|
|
|
|
|
|
|
|
A |
|
U |
||||||||||||
|
|
|
|
|
|
|
|
|
C |
|
|
|
|
|
|
A |
|
|
|
|
|
||
|
|
|
|
|
|
|
|
U |
|
|
|
|
|
|
|
A |
|
|
|
|
|
U U U
RNA, the base pairing is adenine-uracil (A-U) instead of adenine-thymine (A-T). Also the backbone sugars are ribose rather than deoxyribose, but are still linked by phosphate groups. The RNA is very difficult to synthesize as a single crystal and consequently there is a very limited amount of X-ray diffraction data available for RNA. Little is known about its variations, and therefore direct investigations of single molecule RNA would add much to our knowledge.
Transfer RNA is a small RNA chain of 74–93 nucleotides that transfers a specific amino acid to a growing polypeptide chain at the ribosomal site of protein synthesis during translation (15). It has sites for amino acid attachment, and an anticodon region for codon recognition that binds to a specific sequence on the messenger RNA (m- RNA) chain. It has a partial double-helix structure even though it has only one chain, because the single RNA chain folds back, and loops back, on itself, as shown in Fig. 4a.
X-ray diffraction studies (16) have indicated that the t-RNA structure may often assume an L-shaped conformation with a long and a short arm. A model of the Escherichia Coli lysine t-RNA macromolecule used by the group for its STM studies is shown in Fig. 4a and b. It shows both the L conformation and the underlying loop and base pair chemistry.
Using STM, the group was able to directly image the L conformation as shown in Fig. 4c. In addition to the first direct statistical data on the lengths of the long and short arms, obtained from analysis of several STM images, an analysis of the influence of pH on conformation was also carried out. Current investigations are focusing on biofunction research issues in addition to structural research issues, using STM to directly image the coupling of the important amino acid molecules at specific t-RNA sites.
The STM investigations of nanobiomedical rather than structural issues are an important emerging research area. One example is the recently reported research from the University of Sydney group in which the local binding of retinoic acid, a potent gene regulatory molecule, to plasmid p-GEM-T easy (596 base pair Promega) DNA fragments on a single-crystal graphite substrate, was directly imaged and reported (17). Retinoic acid has been documented as responsible for a number of profound effects in cell differentiation and proliferation, and is known to accomplish its functions through selective site binding during the transcription process. The STM images of retinoic acid by itself
(c)
Figure 4. (a) Model of t-RNA L-shaped conformation. (b) Model base pair arrangement in L-shaped conformation. (c) STM image of L- shaped conformation at physiological pH. (Reproduced from Ref. 10, used with permission.)
19.5 nm
on a single-crystal graphite substrate were investigated first. These showed sizes consistent with the retinoic acid molecular structure, and a bright head area with a darker tail area. A molecular model of retinoic acid, also shown in Fig. 5a, shows its aliphatic carbon ring head and polymeric tail. For reasons further discussed below, the aliphatic ring head may be expected to have a higher tunneling current associated with it than the polymeric tail, and therefore the observed bright and dark areas are consistent with the expected structure.
At low concentrations, retinoic acid was observed to bind selectively at minor groove sites along the DNA, with some clustering of retinoic acid molecules observed, as shown in Fig. 5b. High resolution STM imaging provided direct evidence for alignment of the retinoic acid molecules head-to-tail structure edge-on with the minor groove and also in steric alignment with each other. From STM height studies, it could also be inferred that the aliphatic ring head was attached to a ring partner along the minor groove surface, but that the tail was not attached. This may suggest a loosely bound on–off functional mechanism. At high concentrations, retinoic acid was observed to bind along the whole length of the DNA double helix, but again selecting the minor grooves. These first direct studies of selective site binding of retinoic acid with the minor groove of DNA should serve as a template for further direct investigations of other known minor groove binders, thereby opening up the direct investigation of an entire
Figure 5. (a) STM image of retinoic acid on a graphite substrate compared with its molecular model showing the aliphatic ring head and polymeric tail. (b) STM image of retinoic acid binding to t-RNA with molecular model overlay. (Reproduced from Ref. 13, used with permission.)
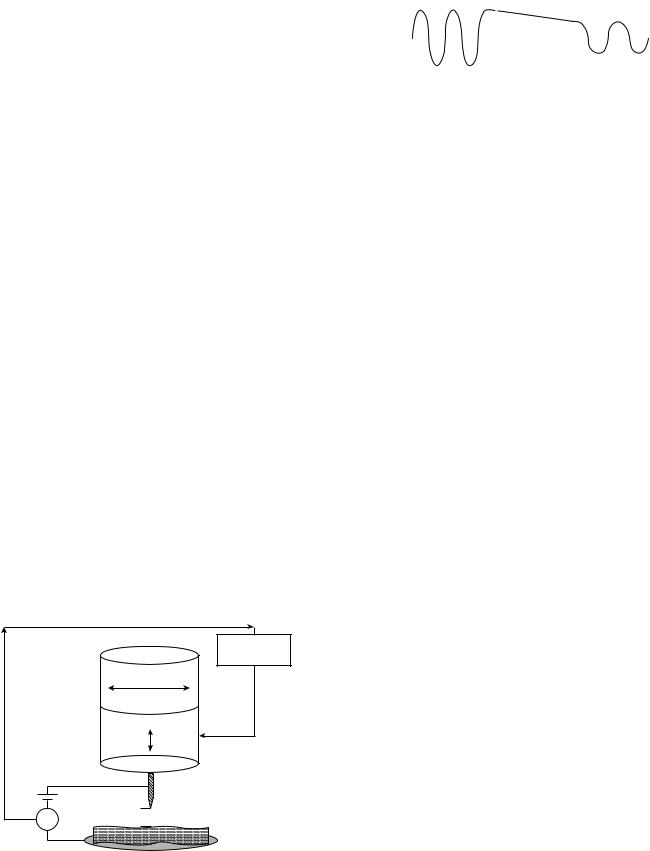
class of regulatory molecule–DNA interactions. The interactions of related structures that are candidate therapeutic drug molecules could be receive similar direct investigation.
Note that both of the above groups have also made important contributions to sample preparation techniques for successful STM analysis of DNA and RNA. These sample preparation techniques will be discussed below in the context of the basic physics of the STM interaction, and the basic chemistry and conductivity of DNA and RNA samples.
BASIC PHYSICS OF THE STM INTERACTION
The STM is based on tip–sample interaction via a tunneling current between filled electronic states of the sample (or tip) into the empty electronic states of the tip (or sample), in response to an applied bias, as shown in Fig. 6. The bias may be positive or negative, and different and valuable information may often be obtained by investigation of the how the sample behaves in accepting, as well as in giving up, electrons. In STM imaging, it is important to recognize that the feature map or apparent topography of the acquired image is really a map of the local density of electronic states. Bright does not correspond to a raised topography; it corresponds to a region with a high density of electronic states. Therefore, in STM imaging of biological samples, an important consideration is that a differential conductivity will be observed from regions, such as rings (usually high) versus regions, such as alkane backbones (usually low). As in all SPM techniques, a z-direction feedback loop maintains some aspect of the tip samples interaction constant (Fig. 6). The readily available choices on commercial machines are to hold either the tunneling distance d constant (constant height mode) or the magnitude of the tunneling current content (constant current mode).
The current in question is a tunneling current, which is a quantum mechanical phenomenon. It is well documented that all electrons within the atomic planes of any material are in fact in such tight quarters that they display the characteristics of a wave in a waveguide, in addition to
Feedback loop |
Controller |
|
|
|
|
|
scanner |
electronics |
|
X, Y |
|
|
Piezoelectric |
|
|
Z |
|
|
|
|
V |
I ~ V exp–c d |
Tip |
|
||
A |
|
d |
Figure 6. Important features of an STM system.
MICROSCOPY, SCANNING TUNNELING |
519 |
|||||
|
|
|
|
|
||
Electron |
|
Tunneling Current |
|
|
|
|
energy is less |
|
|
I ~ V exp–c d |
|
|
|
than barrier |
|
|
|
|
||
energy |
|
|
|
|
|
|
|
|
|
|
|
|
|
0 |
d |
|
|
Figure 7. A particle penetrating into and through a wall.
their particle-likeness. An electron at the surface of a material faces a wall (barrier) created by the dissimilar material (e.g., air, vacuum, or a liquid). While a particle would run into a wall and bounce back, a wave can penetrate into and indeed through a wall (as light goes through glass). This is illustrated in Fig. 7. Additionally, all materials have precise energy levels within them, and therefore, electrons will move by going from one energy level at location 0 to another at location d, meeting conservation of energy requirements.
In STM, a tip with empty electronic states is brought physically close to a sample surface. The electrons are given a direction through the application of the bias (positive in this example). Because they are wavelike, when they reach the sample surface, they can tunnel through the barrier created by the 0-to-d gap and reach the empty states of the tip, where they are recorded as a current proceeding from sample to tip. A tunneling current has the known mathematical form: I V exp cd, where I is the tunneling current, V is the bias voltage between the sample and the tip, c is a constant and d is the tip-sample separation distance. The tunneling current depends sensitively on the size of the 0-to-d gap distance. To observe a tunneling current, the gap must be on the order of tens of nanometers. This is the case in any commercial STM system. It is remarkable, that with the addition of a simple feedback loop, a tip can be easily maintained within nanometers of a sample surface without touching it. Typical STM tunneling currents are on the order of 10 9–10 12 A. With special preamplifiers, currents on the order of 10 14 A can be detected.
Because STM is a current-based technique, some situations that can interfere with its current will be briefly discussed. Very common in STM imaging of biological samples is for the tip to acquire a layer of biological material, possibly by going too close to the sample surface while passing over an insulating region where the feedback loop has little to work on. This usually just introduces image artifacts, discussed below, but it can sometimes insulate the tip from the sample, thus terminating the tip–sample interaction. The problem can be minimized through careful consideration of the expected chemistry and local conductivity of the biological specimen to be investigated.
CHEMISTRY, CONFORMATION, AND CONDUCTIVITY OF BIOLOGICAL SAMPLES
Consideration of the basic chemistry involved in a biological sample can help to determine its appropriateness for STM imaging. The building blocks for DNA and RNA are
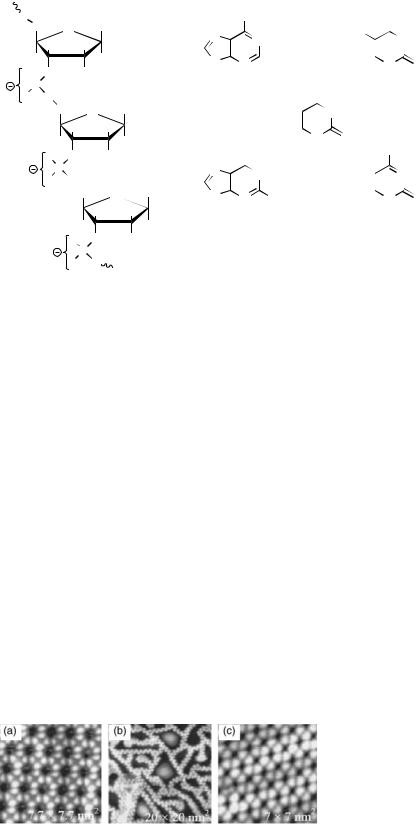
520 |
MICROSCOPY, SCANNING TUNNELING |
|
|
|
|
|
|
|
|
|
|
||||||||
|
|
|
(a) |
|
|
|
|
|
|
|
(b) |
|
|
|
|
|
|
||
O |
CH2 |
|
Base |
|
|
|
NH2 |
|
|
|
|
|
|
O |
|
||||
|
O |
|
N |
|
|
|
N |
|
|
|
|
CH3 |
|
|
|
NH |
|||
|
H |
H |
|
|
|
|
|
|
|
|
|
|
|
||||||
|
|
|
|
|
|
|
|
|
|
|
|
|
|
|
|||||
|
|
|
|
|
|
|
|
|
|
|
|
|
|
|
|
|
|
||
O |
H |
|
H |
|
N |
|
N |
|
|
|
|
|
|
N |
O |
||||
|
|
|
|
|
|
|
|
|
|||||||||||
O |
|
H |
|
|
|
|
|
|
|
|
|||||||||
|
|
H |
|
|
|
|
|
|
|
||||||||||
|
|
|
|
|
|
|
|
|
|
|
|
H |
|
||||||
|
P |
|
|
|
|
|
|
|
|
|
O |
|
|
|
|||||
|
|
|
|
ADENINE |
|
|
THYMINE |
|
|||||||||||
O |
O |
|
|
|
|
|
|
|
|
|
|||||||||
|
|
|
|
|
|
|
|
|
|||||||||||
|
|
|
|
|
|
|
|
|
|
|
|
|
|
||||||
|
|
CH2 |
O |
Base |
|
|
|
|
|
|
|
|
|
NH |
|
|
|
|
|
|
|
|
|
|
|
|
|
|
|
|
|
|
|
|
|
||||
|
|
H |
H |
H |
|
|
|
|
|
|
N |
O |
|
|
|
|
|
||
|
|
|
|
|
|
|
|
|
|
|
|
|
|||||||
|
|
H |
|
|
|
|
|
|
|
|
|
|
|
|
|||||
|
|
|
|
|
O |
|
H |
|
|
NH2 |
|
||||||||
|
O |
O |
H |
|
|
|
|
|
|
|
|||||||||
|
|
|
|
|
|
|
URACIL |
|
|
|
|
|
|||||||
|
|
P |
|
|
N |
|
|
|
NH |
|
|
|
N |
|
|||||
|
|
|
|
|
|
|
|
|
|
|
|||||||||
|
O |
O |
|
|
|
|
|
|
|
|
|
|
|
|
|
|
|
||
|
|
|
|
|
|
|
|
|
|
|
|
|
|
|
|
|
|
||
|
|
|
CH2 O |
Base |
N |
|
N NH2 |
|
|
|
|
|
|
N |
O |
||||
|
|
|
H |
H |
H |
|
|
|
|
|
|
|
|||||||
|
|
|
|
|
|
|
|
|
|
|
|
|
H |
|
|||||
|
|
|
|
|
|
|
|
|
|
|
|
|
|
|
|||||
|
|
O |
H |
H |
GUANINE |
|
|
|
|
CYTOSINE |
|
||||||||
|
|
O |
H |
|
|
|
|
|
|
|
|
|
|
|
|
|
|
|
|
|
|
|
P |
|
|
|
|
|
|
|
|
|
|
|
|
|
|
|
|
|
|
O |
O |
|
|
|
|
|
|
|
|
|
|
|
|
|
|
|
|
Figure 8. (a) The deoxyribose (ribose) sugar/ phosphate backbone for DNA (RNA) is negatively charged due to phosphate groups.
(b) DNA and RNA bases are nitrogenous ring systems.
shown Fig. 8. The sugar-phosphate backbone contains negatively charged phosphate groups for both DNA and RNA. The bases adenine, thymine, uracil, guanine, and cytosine are all nitrogenous ring systems. Thymine, cytosine, and uracil are six-member ring pyrimidine systems, and adenine and guanine are purines, the fusion of a sixmember pyrimidine ring to a five-member imidazole ring. Successful STM imaging of monolayers of the individual bases has been reported (18,19). Examples of the high resolution STM imaging that is possible for monolayers of the individual bases are shown in Figs. 9 and 10.
The nitrogenous ring systems, like the classic benzene ring system, which has also been imaged (20), contain p- orbital electrons above and below the ring structure plane, which create a conductive electron cloud. Hence, the successful STM imaging of the DNA and RNA systems by the Osaka University and University of Sydney groups might be expected from the charged phosphate groups in the backbones and the ring systems in the base pairs.
However, there are also very difficult issues to resolve in making the local conductivity of, especially, the signature DNA and RNA base pairs available to the STM tip. These are enclosed within the sugar-phosphate backbones, and only partially exposed by the twisting of the helix, as shown in Fig. 11a and b (21,22). Also, the choice of substrate will powerfully influence the molecular structure deposited on it, especially if it is small. An example of this is shown in Fig. 12, taken from Ref. 16. The behaviors of pyridine (a
Figure 9. STM images of (a) guanine, (b) cytosine, and (c) adenine monolayers on a single crystal (111)-orientation gold substrate. (Reproduced from Ref. 14, used with permission.)
single-nitrogen close relation to pyrimidine) and benzene on a single crystal (001) orientation copper, Cu(001), substrate were investigated. The pyrimidine monolayers (thymine, cytosine, and uracil) in Figs. 9 and 10 had rings oriented parallel to the substrate surface, but individual pyridine molecules on Cu(001) had rings perpendicular to the surface, due to the strong nitrogen-copper atom interaction, as shown in Fig. 12a. Also, if a single hydrogen atom was dissociated from the pyridine molecule, as can happen during routine scanning, the molecule would shift its position on the copper substrate (Fig. 12b). The STM imaging of an individual benzene molecule indicated a ring system parallel to the copper substrate (Fig. 12c), but hydrogen dissociation would cause the benzene molecule to become perpendicular to the substrate surface (Fig. 12d). Therefore both the substrate choice and interactions with the imaging tip can influence the conformation of the biomolecule and whether its locally conductive portions are positioned to produce a tunneling current.
Now consider the situation of a molecule with a difference in local conductivity, like retinoic acid. The aliphatic ring head would similarly be expected to have a high local conductivity, and separate investigations of just retinoic acid by the University of Sydney group confirmed that this is the case (Fig. 5a). The polymeric tail is basically an alkane system without any p-type orbitals. Its conductivity is therefore expected to be less than the ring system and this is experimentally observed. However, results such as those shown in Fig. 13 from a group at California Institute of Technology, demonstrate that high resolution STM imaging even of low conductivity alkane systems is possible (23–26). Therefore, one aspect of STM biomolecular imaging is that there may be large differences in the conductivities of two closely adjacent regions. It then becomes an issue of whether the STM feedback loop will be able to sufficiently respond to the differences to maintain the tipsample tunneling current interaction throughout the investigation. Prior consideration of the imaging parameters necessary for successful STM imaging of the least conductive part of the bio molecule can help.
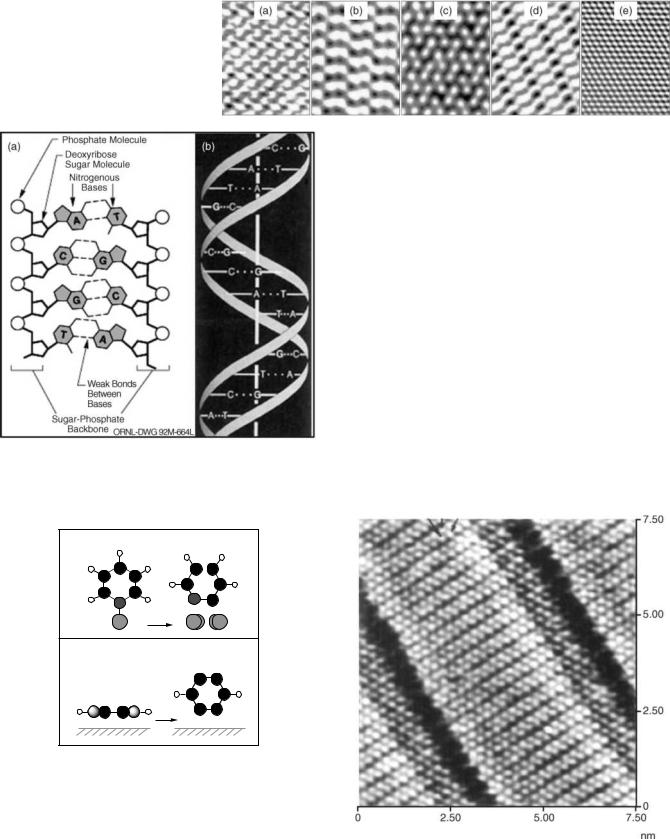
MICROSCOPY, SCANNING TUNNELING |
521 |
Figure 10. STM images of (a) guanine, (b) adenine, (c) uracil, and (d) thymine monolayers on (e) a single crystal (0001)-orientation molybdenum dissulfide substrate. (Adapted from Ref. 15, used with permission.)
Figure 11. The three-dimensional conformation of DNA. (a) The base pairs are positioned between the sugar-phosphate backbones.
(b) The overall structure is a double helix. (Reproduced from Refs. 17,18, used with permission.)
(a) |
Pyridine on Cu(001) |
(b) |
|
|
–H
(c) |
(d) |
|
Benzene on Cu(001) |
–2H
Figure 12. Influence of the sample-substrate interaction on sample orientation. (a) An individual pyridine molecule on a copper (001)-orientation, (Cu(001)) substrate is perpendicular to the surface due to the strong nitrogen–copper atom interaction. (b) An individual pyridine molecule from which a hydrogen atom has dissociated is also perpendicular to a Cu(001) surface but has a shifted location. (c) An individual benzene molecule on a Cu(001) substrate is parallel to the surface but (d) may become perpendicular if hydrogen dissociation occurs. (Adapted from Ref. 16, used with permission.)
Biomolecules, with only nanometer dimensions, always should be deposited on atomically flat single-crystal substrates. Substrates can also be selected to supply electrons to the biomolecule, for positive bias scanning, or to manipulate the biomolecule into a desired position. Another important sample preparation issue is that biomolecules often have multiple available conformations, including globular conformations that self-protect the molecule under nonphysiological conditions. While STM imaging may be performed in vacuum, air, and even in a liquidfilled compartment (liquid cell), the best resolution may be achieved in vacuum, which is a nonphysiological condition. The less physiological the imaging conditions, the more it will be necessary to use special molecular stretching techniques to investigate an open conformation. A special pressure jet injection technique was developed by the Osaka University group to deposit stretched DNA and RNA on single-crystal copper for vacuum STM imaging, without giving them the chance to close into globular conformations (13,14).
Figure 13. High resolution STM images of an alkane (pentatracontane) monolayer on graphite. (Reproduced from Ref. 19, used with permission.)
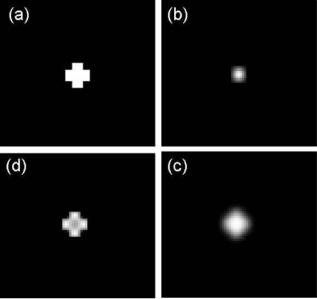
522 MICROSCOPY, SCANNING TUNNELING
IMAGING ARTIFACTS AND DATA RESTORATION USING DECONVOLUTION
Examination of Fig. 5a shows the ring head of retinoic acid as a large blurred bright spot. Greater resolution of detail would clearly be desirable. As in all SPM imaging systems, tip artifacts versus the surface features will limit the resolution of the experiments performed. This is often cited as an ultimate barrier in STM studies of macromolecular structures and in scanning probe microscopy in general (27). It is therefore necessary to develop techniques for deconvolution of STM tip artifacts for enhancing the resolution of measured STM image data.
A commonly used approach for data restoration or eliminating the smearing effect of tip sample interaction is to assume that the observed signal is a convolution of the true image and the probe response function (PRF). The following equation gives a general degradation model due to the convolution of tip artifacts with true data resulting in the measurement g(x,y). Neglecting the presence of the additive noise, the data can be modeled as
X
gðx; yÞ ¼ f ðx; yÞ hðx; yÞ ¼ f ðn; mÞhðx n; y mÞ
n;m
where g(x, y), f(x, y), and h(x,y) are the observed or raw signal, true image, and PRF, respectively. One can then use deconvolution methods to extract the true image from the knowledge of measured data and probe PRF.
Theoretically, the probe response function is derived from the underlying physics of the tip sample interaction process. Hence, there is a need for a theoretical model for the tip sample interaction. Recent advances in formulation and modeling of tip sample interactions allow development of accurate compensation algorithms for deconvolving the effect of tip-induced artifacts.
Figure 14 shows an example of applying a deconvolution algorithm on synthetic degraded images. The degraded image in Fig. 14c is generated from a synthetic image in Fig. 14a blurred by a Gaussian PRF in Fig. 14b. Figure 14d shows the enhanced result obtained using deconvolution. Although the theoretical treatment of STM and related SPM techniques provide major challenges because the atomic structures of the tip and sample have to be modeled appropriately, its potential is clear and this is a strongly developing research area at the present time.
CONCLUSIONS
The STM imaging has the highest resolution of all SPM imaging techniques. As such, it would be highly desirable to apply STM to investigations of molecular biology and medicine. An often described difficulty when considering the application of STM to molecular biology is that biological samples are nonconductive. It would be more accurate to describe biological samples as having both local and varying conductivities. Design of STM experiments in which ring systems are exploited, and/or imaging parameters are set for the least conductive portion of the biomolecules may help produce successful imaging results. New research in applications of powerful deconvolution
Figure 14. Clockwise from upper left: (a) synthetic true image
(b) Gaussian PRF, (c) degraded measurement, and (d) restored image.
techniques to STM imaging will also open up the field of direct STM investigations of the structure and function of important biomolecules.
BIBLIOGRAPHY
1.Binning G, Rohrer H. Helv Phys Acta 1982;55:726–735.
2.Hansma HG, Oroudjev E, Baudrey S, Jaeger L. TectoRNA and kissing loops: Atomic force microscopy of RNA structures. J Microsc 2003;212:273–279; Sitko JC, Mateescu EM, Hansma HG. Sequence-dependent DNA condensation and the electrostatic zipper. Biophys J 2003;84:419–431; Hansma HG, Vesenka J, Siegerist C, Kelderman G, Morrett H, Sinsherimer RL, Bustanmante C, Elings V, Hansma PK. Reproducible imaging and dissection of plasmid DNA under liquid with the atomic force microscope. Science 1992;256:1180.
3.Hartmann U. Magnetic force microscopy. Annu Rev Mater Sci 1999;29:53–87.
4.Paesler MA, Moyer PJ. Near-Field Optics: Theory, Instrumentation, and Applications. New York: Wiley-Interscience; 1996.
5.Majumdar A. Scanning thermal microscopy. Annu Rev Mater Sci 1999;29:505–585.
6.Beebe TP, Jr., et al. Science 1989;243:370–371.
7.Lee G, Arscott PG, Bloomfiled VA, Evans DF. Science 1989;244:475–478.
8.Driscoll RJ, Youngquist MG, Baldescwieler JD. Atomic scale imaging of DNA using scanning tunnelling microscopy. Nature (London) 1990;346:294–296.
9.Tanaka H, Kawai T. Visualization of detailed structures within DNA. Surface Sci Lett 2003;539:L531–L536.
10.Nishimura M, Tanaka H, Kawai T. High resolution scanning tunneling microscopy imaging of Escherichia coli lysine transfer ribonucleic acid. J Vac Sci Technol B 2003;21:1265–1267.
11.Wikipedia, The free encyclopedia. Available at http://en.wikipedia.org/wiki/RNA.