
- •VOLUME 4
- •CONTRIBUTOR LIST
- •PREFACE
- •LIST OF ARTICLES
- •ABBREVIATIONS AND ACRONYMS
- •CONVERSION FACTORS AND UNIT SYMBOLS
- •HYDROCEPHALUS, TOOLS FOR DIAGNOSIS AND TREATMENT OF
- •HYPERALIMENTATION.
- •HYPERBARIC MEDICINE
- •HYPERBARIC OXYGENATION
- •HYPERTENSION.
- •HYPERTHERMIA, INTERSTITIAL
- •HYPERTHERMIA, SYSTEMIC
- •HYPERTHERMIA, ULTRASONIC
- •HYPOTHERMIA.
- •IABP.
- •IMAGE INTENSIFIERS AND FLUOROSCOPY
- •IMAGING, CELLULAR.
- •IMAGING DEVICES
- •IMMUNOLOGICALLY SENSITIVE FIELD–EFFECT TRANSISTORS
- •IMMUNOTHERAPY
- •IMPEDANCE PLETHYSMOGRAPHY
- •IMPEDANCE SPECTROSCOPY
- •IMPLANT, COCHLEAR.
- •INCUBATORS, INFANTS
- •INFANT INCUBATORS.
- •INFUSION PUMPS.
- •INTEGRATED CIRCUIT TEMPERATURE SENSOR
- •INTERFERONS.
- •INTERSTITIAL HYPERTHERMIA.
- •INTRAAORTIC BALLOON PUMP
- •INTRACRANIAL PRESSURE MONITORING.
- •INTRAOCULAR LENSES.
- •INTRAOPERATIVE RADIOTHERAPY.
- •INTRAUTERINE DEVICES (IUDS).
- •INTRAUTERINE SURGICAL TECHNIQUES
- •ION-EXCHANGE CHROMATOGRAPHY.
- •IONIZING RADIATION, BIOLOGICAL EFFECTS OF
- •ION-PAIR CHROMATOGRAPHY.
- •ION–SENSITIVE FIELD-EFFECT TRANSISTORS
- •ISFET.
- •JOINTS, BIOMECHANICS OF
- •JOINT REPLACEMENT.
- •LAPARASCOPIC SURGERY.
- •LARYNGEAL PROSTHETIC DEVICES
- •LASER SURGERY.
- •LASERS, IN MEDICINE.
- •LENSES, CONTACT.
- •LENSES, INTRAOCULAR
- •LIFE SUPPORT.
- •LIGAMENT AND TENDON, PROPERTIES OF
- •LINEAR VARIABLE DIFFERENTIAL TRANSFORMERS
- •LITERATURE, MEDICAL PHYSICS.
- •LITHOTRIPSY
- •LIVER TRANSPLANTATION
- •LONG BONE FRACTURE.
- •LUNG MECHANICS.
- •LUNG PHYSIOLOGY.
- •LUNG SOUNDS
- •LVDT.
- •MAGNETIC RESONANCE IMAGING
- •MAGNETOCARDIOGRAPHY.
- •MANOMETRY, ANORECTAL.
- •MANOMETRY, ESOPHAGEAL.
- •MAMMOGRAPHY
- •MATERIALS, BIOCOMPATIBILITY OF.
- •MATERIALS, PHANTOM, IN RADIOLOGY.
- •MATERIALS, POLYMERIC.
- •MATERIALS, POROUS.
- •MEDICAL EDUCATION, COMPUTERS IN
- •MEDICAL ENGINEERING SOCIETIES AND ORGANIZATIONS
- •MEDICAL GAS ANALYZERS
- •MEDICAL PHOTOGRAPHY.
- •MEDICAL PHYSICS LITERATURE
- •MEDICAL RECORDS, COMPUTERS IN
- •MICROARRAYS
- •MICROBIAL DETECTION SYSTEMS
- •MICROBIOREACTORS
- •MICRODIALYSIS SAMPLING
- •MICROFLUIDICS
- •MICROPOWER FOR MEDICAL APPLICATIONS
- •MICROSCOPY AND SPECTROSCOPY, NEAR-FIELD
- •MICROSCOPY, CONFOCAL
- •MICROSCOPY, ELECTRON
- •MICROSCOPY, FLUORESCENCE
- •MICROSCOPY, SCANNING FORCE
- •MICROSCOPY, SCANNING TUNNELING
- •MICROSURGERY
- •MINIMALLY INVASIVE SURGICAL TECHNOLOGY
- •MOBILITY AIDS
- •MODELS, KINETIC.
- •MONITORING IN ANESTHESIA
- •MONITORING, AMBULATORY.
- •MONITORING, FETAL.
- •MONITORING, HEMODYNAMIC
- •MONITORING, INTRACRANIAL PRESSURE
- •MONITORING, NEONATAL.
- •MONITORING, UMBILICAL ARTERY AND VEIN
- •MONOCLONAL ANTIBODIES
- •MOSFET.
- •MUSCLE ELECTRICAL ACTIVITY.
- •MUSCLE TESTING, REHABILITATION AND.
- •MUSCULOSKELETAL DISABILITIES.
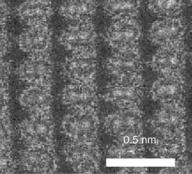
478 MICROSCOPY, ELECTRON
MICROSCOPY, ELECTRON
MAHROKH DADSETAN
LICHUN LU
MICHAEL J. YASZEMSKI
Mayo Clinic,
College of Medicine
Rochester, Minnesota
INTRODUCTION
Invention of the light microscope by Janssens in 1590 was the first milestone in the microscopic world. Janssens’ microscope magnified objects up to 20–30 times their original size. By the beginning of the twentieth century, objects could be magnified only up to 1000 times with a resolution of 0.2 mm. In the early 1930s, the limitations of light microscopes and the scientific desire to see intracellular structural details, such as mitochondria and nuclei led to the development of electron microscopes. The electron microscope took advantage of the much shorter wavelength of the electron compared to that of visible light. With the electron microscope, another 1000-fold increase in magnification was accomplished with a concomitant increase in resolution, allowing visualization of viruses, deoxyribonuclic acid (DNA), and smaller objects, such as molecules and atoms. The transmission electron microscope (TEM) was the first type of electron microscope, and was developed by Ruska and Knoll in Germany in 1931. Electron microscopy is based on a fundamental physics concept stated in the de Broglie theory (1924). This concept is that moving electrons have the properties of waves. The second major advancement in electron microscopy was made by Busch, who demonstrated in 1926 that electrostatic or magnetic fields could be used as a lens to focus an electron beam. In 1939, Siemens Corp. began commercial production of a microscope developed by Von Borries and Ruska in Germany. Hiller, Vance and others constructed the first TEM in North America in 1941. This instrument had a resolution of 2.5 nm.
About the same time that the first TEM was nearing completion in the 1930s, a prototype of the scanning electron microscope (SEM) was constructed by Knoll and Von Ardenne in Germany. However, the resolution of this microscope was no better than that of the light microscope. Following several improvements made by RCA in the United States, as well as Cambridge University in England, a commercial SEM became available in 1963. A later version of the SEM made by the Cambridge Instrument Co. had a resolving power of 20–50 nm and a useful magnification of 75,000 . Recent models of the SEM have a resolving power of 3.0 nm and magnifications up to 300,000 .
Although the design of TEM and SEM is similar in many ways, their applications are very different. The TEM is patterned after as light microscope, except that electrons instead of light pass through the object. The electrons are then focused by two or more electron lenses to form a greatly magnified image onto photographic film or a charge coupled device (CCD) camera. The image produced by TEM is two-dimensional (2D) and the brightness of a particular
region of the image is proportional to the number of electrons that are transmitted through the specimen at that position on the image. The SEM produces a three-dimensional (3D) image by scanning the surface of a specimen with a 2–3 nm spot of electrons to generate secondary electrons from the specimen that are then detected by a sensor. The resolution of an SEM is limited by two quite different sets of circumstances. One of these is concerned with the physics of electron optics, while the other depends on the penetration of electrons into the object being imaged.
A third type of electron microscope, the scanning transmission electron microscope (STEM) has features of both the transmission and scanning electron microscopes. This microscope is an analytical tool that determines the presence and distribution of the atomic elements in the specimen. Recently, two groups of researchers have accomplished a subangstrom resolution (0.06 nm) for STEM using an aberration corrector. They have reported that columns of atoms in a silicone crystal that are 0.078 nm apart can be distinguished at this resolution (1). The image of Si shown in Fig. 1 has been recorded in a high angle annular dark field (HAADF) mode, and the pairs of atomic columns are seen directly resolved. The HAADF detector collects electrons scattered by the sample to angles greater than the detector inner radius. Such high angle scattering is largely incoherent thermal diffuse scattering, which means that the resolution observed in the image is determined by the intensity distribution of the illuminating probe. With this advantage over conventional coherent high resolution transmission electron microscopy (HRTEM), HAADF–STEM has enabled imaging not only of individual atomic columns in crystals, but single dopant atoms on their surface and within their interior.
In 1982, another type of electron microscope, the scanning tunneling microscope (STM) was developed by two scientists, Rohrer and Binnig, for studying surface structure. This invention was quickly followed by the development of a family of related techniques classified as scanning probe microscopy (SPM). These techniques are based upon moving a probe (typically called a tip in STM, which is literally a sharp metallic object) just above a specimen’s surface while monitoring some interaction
Figure 1. Image of a silicone crystal observed in the [112] orientation recorded with an aberration corrected STEM. (From Ref. 1). Reproduced by courtesy of American Association for the Advancement of Science.)
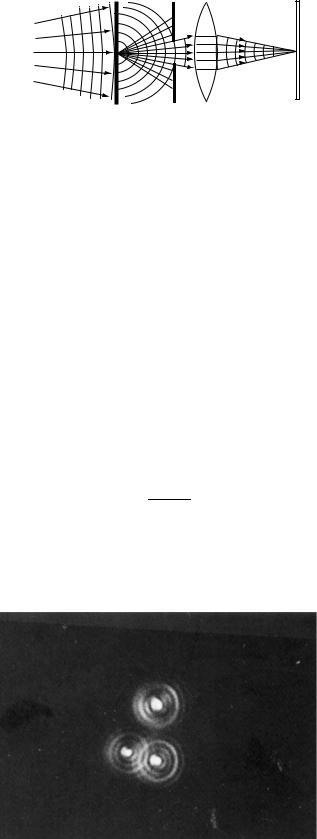
between the probe and the surface. Atomic force microscopy (AFM) is another important technique of this kind but is not categorized as electron microscopy. Bennig and Rohrer were awarded one-half of the 1986 Nobel Prize in physics for invention of the STM, while Ernst Ruska was awarded the other one-half of that same Nobel Prize for his 1931 invention of the electron microscope. In STM, electrons are speeded up in a vacuum until their wavelength is extremely short, only one hundred-thousandth that of white light. Beams of these fast-moving electrons are focused on a cell sample and are absorbed or scattered by the cell’s parts so as to form an image on an electronsensitive photographic plate. The STM is widely used in both industrial and academic research to obtain atomic scale images of metal surfaces. It provides a 3D profile of the surface, which is useful in characterizing surface roughness and determining the size and conformation of surface molecules. (2) Invention of electron microscopy had an enormous impact in the field of biology, specifically in cell and tissue analysis. Almost 15 years after the invention of the first electron microscope by Ruska, many efforts were made to apply this technique to biological problems. Using the electron microscope, cell organelles and cell inclusions were discovered or resolved in finer details. Electron microscopy, specifically TEM, is now among the most important tools in cell biology and diagnostic pathology.
The latest advancement in electron microscopy is 3D reconstruction of cellular components at a resolution that is on the order of magnitude of atomic structures defined by X-ray crystallography. The method for reconstruction of 3D images of single, transparent objects recorded by TEM is called electron tomography (ET). In order to generate 3D images of individual molecules, one needs to obtain as many tilt images as possible, covering the widest possible angular range. The representative images of particles obtained from different orientations is then analyzed and combined by a software program to reconstruct the molecule in 3D. With improvements in instrumentation, data collection methods and techniques for computation, ET may become a preferred method for imaging isolated organelles and small cells. So far, the electron tomography method covers the resolution range of 2.5–5.0 nm. Data obtained via electron tomography furnish a rich source of quantitative information about the structural composition and organization of cellular components. It offers the opportunity to obtain 3D information on structural cellular arrangements with a significantly higher resolution than that provided by any other method currently available (e.g., confocal laser microscopy) (3).
THEORY OF ELECTRON MICROSCOPY
According to electromagnetic theory, a light source initiates a vibrational motion that transmits energy in the direction of propagation. The wave motion of light is analogous to that produced by a stone thrown into a pool of water. When the waves generated from throwing a stone strike an object that has an opening or aperture, another series of waves is generated from the edge of the object. The result is a new source of waves that emerges with the
MICROSCOPY, ELECTRON |
479 |
Light |
|
|
|
from |
|
|
|
distant |
|
|
|
source |
|
|
|
Pinhole |
Circular |
Biconcave |
Viewing |
aperture |
limiting |
lens |
screen |
|
aperture |
|
|
Figure 2. Diffraction of light waves.
original waves. This bending or spreading phenomenon is known as diffraction. Diffracted waves interfere with the initial waves, and the result is an image of the edge of the object. The edge appears to have a series of bands or fringes called Fresnel fringes running parallel to the edge (Fig. 2). Thus, if a strong beam of light illuminates a pinhole in a screen and thus a pinhole serves as a point source and the light passing through is focused by an apertured ‘‘perfect’’ lens on a second screen, the image obtained is not a pinpoint of light, but rather a bright central disk surrounded by a diffuse ring of light. Even if monochromatic light was used to illuminate the point source and was to pass through a perfect lens, the image will not be a sharp one, but rather a diffuse disk composed of concentric rings. This type of image is known as an Airy disk after Sir George Airy, who first described this pattern during the nineteenth century (Fig. 3) (4). To determine resolving power (RP), it is important to know the radius of the Airy disk. The radius of the Airy disk as measured to the first dark ring (r) is expressed by following equation:
0:612 l |
ð1Þ |
r ¼ nðsin aÞ |
In Eq. 1, l ¼ wavelength of illumination; n ¼ refractive index of the medium between the point source and the lens, relative to free space; a ¼ half the angle of the cone of light from the specimen plane accepted by the front lens of objective
Figure 3. Airy disks generated by viewing three pinholes in a light microscope. Magnification of micrograph is 1000 . (From Ref. 4. Reproduced by courtesy of Jones and Bartlett Publishers.)
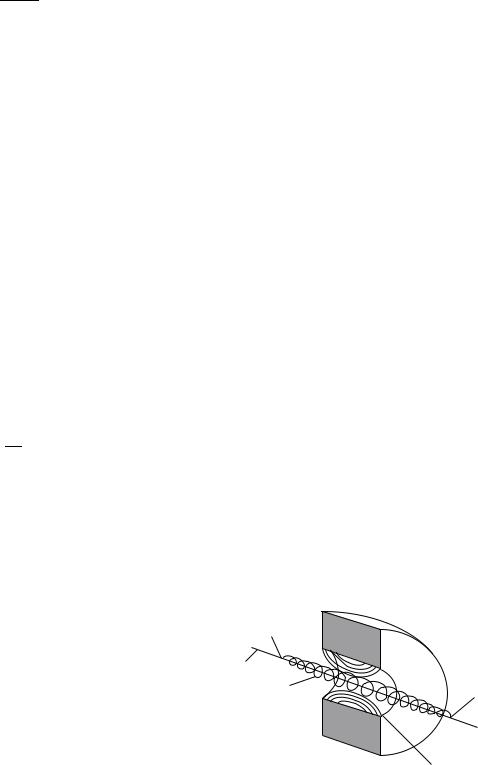
480 MICROSCOPY, ELECTRON
The above equation can be shown in another form as:
d ¼ |
0:612 l |
ð2Þ |
NA |
where NA (numerical aperture) ¼ n sin a and represents the light gathering power of the lens aperture.
From the above equation, RP is defined as the minimum distance that two objects can be placed apart and still be seen as separate entities. Consequently, the shorter the distance, the better (or higher) is the RP of the system. For example, consider a light microscope using ultraviolet (UV) light, which lies beyond the lower end of the visible spectrum (400 nm). Further specifications of this system include a glass slide with standard immersion oil (refractive index, n ¼ 1.5), and sina= 0.87 (sine of a 648 angle, representing one-half of the 1288 acceptance angle of a glass lens. The theoretical resolution that can be attained by this system is 0.2 mm. In other words, two points in the specimen that are not separated by at least this distance will not be seen as two distinct points, but will be observed as a single blurred image. Since the values of sin a and n cannot be significantly increased beyond the stated values, the RP can most effectively be improved by reducing wavelength.
Electron Beams And Resolution
The concept that moving electrons might be used as an illumination source was suggested by a tenet of the de Broglie theory, that moving electrons have wave properties. The wavelength of this particle-associated radiation is given by following equation:
h |
ð3Þ |
l mn |
where m is the mass of the particle, v the velocity of
particle, and h is Planck’s |
constant (6.626 10 34J 1). |
For an electron accelerated |
by a potential of 60,000 V |
(60 kV), the wavelength of the electron beam would be0.005 nm, which is 100,000 times shorter than that for green light. By using Eq. 1, a TEM with perfect lenses would therefore in theory be able to provide a resolution of 0.0025 nm. In practice, the actual resolution of a modern high resolution transmission electron microscope is closer to 0.2 nm. The reason we are not able to achieve the nearly 100-fold better resolution of 0.002 nm is due to extremely narrow aperture angles ( 1000 times smaller than that of the light microscope) needed by the electron microscope lenses to overcome a major resolution limiting phenomenon called spherical aberration. In addition, diffraction, chromatic aberration and astigmatism all contribute to decreased resolution in TEM, and need to be corrected to achieve higher resolution. (5)
Magnification
The maximum magnification of any microscope is simply the ratio of the microscope’s resolution to the resolution of the unaided human eye. The resolution of the eye viewing an object at 25 cm is generally taken to be 0.25 mm. Since the resolution of a light microscope is 0.25 mm, maximum useful light magnification is 1000 , obtainable from an
objective lens of 100 followed by an eyepiece of 10 . The magnification of TEM would be 0.25mm/0.25 nm. This is a 106 magnification, and corresponds to a 1000-fold increase in resolution compared to a light microscope. An objective lens of 100 is followed by an ‘‘intermediate’’ lens of 25 , and the final image is projected by a projector lens of 100 . Further magnification for critical focusing is obtained by viewing the image on the fluorescent screen with a long working distance binocular microscope of 10 . The final image is photographed at 250,000 . The processed negative is then enlarged a further 4 in a photographic enlarger. This result in a final prints (the electron micrograph) at the desired magnification of 106 6.
Electromagnetic Lenses
An electromagnetic lens is generated by a coil of wire with a direct current (dc) that passes through the coil. This electromagnetic coil is called a solenoid. It forms an axially and radially symmetric magnetic field that converges to a point. A divergent cone of electrons enters from a point source, and thus forms a real image on the lens axis. An advantage of electromagnetic lenses is that the focal length can be made infinitely variable by varying the coil current. Therefore, both magnification and image focus can be adjusted by controlling the lens current (Fig. 4) (7).
Lens Aberrations
Electron lenses are affected by all the aberrations of optical lenses, such as spherical aberration, chromatic aberration, astigmatism, and distortion. Spherical aberration results from the geometry of both glass and electromagnetic lenses such that rays passing through the periphery of the lens are refracted more than rays passing along the axis. Spherical aberration may be reduced by using an aperture to eliminate some of the peripheral rays. Although this aperture is attractive for reducing spherical aberration, it decreases the aperture angle and thereby prevents the electron microscope from achieving the theoretical resolution predicted by Eq. 1.
Chromatic aberration results when electromagnetic radiations of different energies converge at different focal
Source of
electrons
Axis
Electron |
Focal |
|
point |
||
trajectory |
||
|
Magnetic lens field
Figure 4. Single electron passing through electromagnetic lens. The electron is focused by the magnetic field to follow a trajectory that will converge at a defined focal point after it emerges from the lens.
planes. Chromatic aberration results in the enlargement of a focal point with a consequential loss of resolution. It can be corrected by using a monochromatic source of electromagnetic radiation. This entails stabilizing the accelerating voltage to generate the electrons with same levels of energy, and having a good vacuum to minimize the energy loss of the electrons during their passage through the transmission specimen. This effect can also be reduced by decreasing the aperture of the objective lens. (6)
Astigmatism is caused by radial asymmetry in a lens, giving rise to a focal length in one plane that is different from that in another plane. The fabrication and maintenance of a lens that has a perfectly symmetric lens field is not feasible in practice. Thus, it is necessary to correct astigmatism by applying a radial symmetry compensator device called a stigmator. This consists of an adjustable electric or magnetic field that can be applied across the lens in any chosen direction, thus compensating for astigmatism. Image distortion, due to magnification changing across the field from the value at the center, may be positive (called barrel distortion) or negative (called pincushion distortion). These effects can be compensated by operating two lenses in series, arranging for barrel distortion in one to be compensated by pincushion distortion in the other. The lens system in modern electron microscopes is designed to automatically counterbalance the various types of distortions throughout a wide magnification range. (4)
DESIGN OF THE TRANSMISSION ELECTRECTRON MICROSCOPE
Both the light and electron microscopes are similar so far as the arrangement and function of their components are concerned. Thus, both microscopes, when used for photographic purposes, can be conveniently divided into the following component systems.
Illuminating System
This system serves to produce the required radiation and to direct it onto the specimen. It consists of source and condenser lenses.
Source Lens. The source of electrons, or cathode, is a hairpin of fine tungsten wire about 2 mm long, maintained at 2500 K by 2 W of alternting current (ac) or dc power. Electrons boil off the white-hot tungsten surface, and are shaped into a conical beam by an electrode system called the gun. Two further electrodes, the shield and the anode, combine to form an electrostatic collimating lens and accelerator. A suitable accelerating voltage (20–100 kV) is chosen for the specimen under examination, and is applied to the cathode as a negative potential so that the anode may remain at earth potential. The cathode and shield are therefore carried on an insulator. The filament-shield voltage (cathode bias) is made variable to adjust the total current drawn from the filament, which in turn varies the brightness of the final image (4).
The energy of the electrons in the TEM determines the relative degree of penetration of electrons into a specific sample, or alternatively, influences the thickness of mate-
MICROSCOPY, ELECTRON |
481 |
rial from which useful information may be obtained. Thus, a high energy TEM (400 kV) not only provides the highest resolution but also allows for the observation of relatively thick samples (e.g., 0.2 mm) when compared with the more conventional 100 kV or 200 kV instruments. Because of the high spatial resolution obtained, TEMs are often employed to determine the detailed crystallography of finegrained, or rare, materials (6,8).
Condenser Lens. The condenser lens regulates the convergence (and thus the intensity) of the illuminating beam on the specimen. The divergent electron beam emerging from the anode aperture can, in simple instruments, be used to illuminate the specimen directly. However, sufficient image brightness for high magnification is difficult to obtain. As in the light microscope, a condenser system is almost invariably interposed between the gun and specimen to concentrate the beam on the region of the specimen under examination. A single condenser lens suffices for electronoptical work up to 50,000 . However, for high resolution work a double condenser system is always used, which will concentrate the beam into an area as small as 1m diameter.
Specimen Manipulation System
The pierced metal grid carrying the specimen proper is clamped at its periphery to a suitable holder designed to conduct heat rapidly away. The specimen temperature in a TEM may rise to 200 8C. The holder, including its attached specimen grid is introduced into the evacuated specimen chamber through an airlock by means of an insertion tool. This tool is then generally withdrawn after the holder has been placed on the translation stage. The holder is then free to move with the stage, which is driven from outside the column through airlocks, by means of levers and micrometer screws. Two mutually perpendicular stage movements, each of about 1 mm, allow any part of the grid area to be brought to the microscope axis and viewed at the highest magnification. Most instruments provide a scan magnification so that the whole grid area may be viewed at100 . Suitable specimen areas are then chosen, and centered for study at higher magnifications.
Imaging System
This part of the microscope includes the objective, intermediate, and projector lenses. It is involved in the generation of the image and the magnification and projection of the final image onto a viewing screen or camera system. Electrons transmitted by the specimen enter the objective lens. Those passing through the physical aperture are imaged at 100 in the intermediate lens object plane, 10–20 cm below the specimen. The position of this primary image plane is controlled by the objective lens current (focus control). A second image, which may be magnified or diminished, is formed by the intermediate lens, the current through which controls overall magnification (magnification control). This secondary image, formed in the objective plane of the projector lens, is then further magnified, and the overall magnification is determined by the position of the fluorescent screen or film.
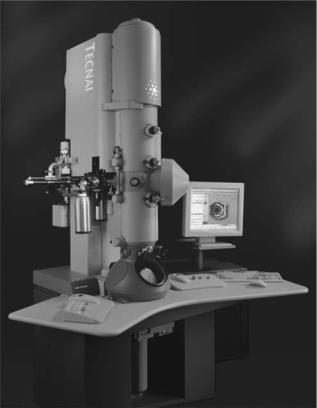
482 MICROSCOPY, ELECTRON
Image Recording System
The final image is projected onto a viewing screen coated with a phosphorescent zinc-activated cadmium sulfide powder. This powder is attached to the screen with a binder, such as cellulose nitrate. Most electron microscopes provide for an inclination of the viewing screen so that the image may be conveniently examined either with the unaided eye or through a stereomicroscope (the binoculars). Although the stereomicroscope image may appear to be rough due to the 100 mm sized phosphorescent particles that make up the screen, it is necessary to view a magnified image in order to focus accurately. Some microscopes may provide a second, smaller screen that is brought into position for focusing. In this case, the main screen remains horizontal, except during exposure of the film. All viewing screens will have areas marked to indicate where to position the image so that it will be properly situated on the film. Preevacuated films are placed into an air lock (camera chamber) under the viewing screen and the chamber evacuated to high vacuum. The chamber is then opened to the column to permit exposure of the film. In modern electron microscopes (Fig. 5), exposure is controlled by an electrically operated shutter placed below the projector lens. As one begins to raise the viewing screen, the shutter blocks the beam until the screen is in the appropriate position for exposure. The shutter is then opened for the proper interval, after which the beam is again blocked until the screen is repositioned.
Figure 5. Image of a 300 kV TEM (FEI-Tecnai G2 Polara) for cryoapplications at liquid nitrogen and liquid helium temperatures. (Reproduced by courtesy of FEI Company.)
DESIGN OF THE SCANNING ELECTRON MICROSCOPE
The SEM is made up of two basic systems, and the specimen is at their boundary. The first system is the electron optical column that provides the beam of illumination that is directed to the specimen. The second system consists of the electron collection, signal amplification, and image display units, which converts the electrons emitted from the specimen into a visible image of the specimen.
Electron Optical Column
The electron gun and electron lenses are present in the electron optical column of the SEM in an analogous fashion to their presence in the TEM.
1.Electron Gun: The electron source is most commonly the hairpin tungsten filament located in a triode electron gun. The electrons are emitted by the filament (also called the cathode), and accelerated by a field produced by the anode. The anode is usually at a positive potential on the order of 15 kV with respect to the cathode. A third electrode, the shield, lies between the anode and cathode and is negative with respect to the cathode. After leaving the bias shield and forming an initial focused spot of electrons of50 mm in diameter, a series of two to three condenser lenses are used to successively demagnify this spot sometimes down to 2 nm. These small spot sizes are essential for the resolutions required at high magnifications. A heated tungsten filament is the conventional electron source for most SEMs; other special sources are lanthanum hexaboride
(LaB6) and the field emission guns (FEG). Both of these latter sources produce bright beams of small diameter and have much longer lifetimes than heated tungsten filaments. Schottky emission has largely replaced earlier source technologies based on either tungsten and LaB6 emission or cold-field emission in today’s focused electron beam equipment including SEM, TEM, Auger systems, and semiconductor inspection tools. Schottky and cold-field emission are superior to thermionic sources in terms of source size, brightness and lifetime. Both are up to 1000 times smaller and up to 100 times brighter than thermionic emitters.
2.Electron Lenses: Most SEMs have three magnetic lenses in their column: the first, second, and final condenser lenses. The first condenser lens begins the demagnification of the 50 mm focused spot of electrons formed in the region of the electron gun. As the amount of current running through the first condenser lens is increased, the focal length of the lens becomes progressively shorter and the focused spot of electrons becomes smaller. In our earlier discussion of electron lenses, it was noted that focusing takes place by varying the focal length. This is accomplished by changing the intensity of the lens coil current, which in turn alters the intensity of the magnetic field that is generated by the lens. As the lens current increases, the lens strength increases
and the focal length decreases. A short focal length lens consequently causes such a wide divergence of the electrons leaving the lens that many electrons are not able to enter the next condenser lens. The overall effect of increasing the strength of first condenser lens is to decrease the spot size, but with a loss of electrons. An aperture is positioned in the lenses to decrease the spot size and reduce spherical aberration by excluding the more peripheral electrons. Each of the condenser lenses behaves in a similar manner and possesses apertures.
In designing the final condenser lens, several performance characteristics must be considered:
(a)Aberrations. Since the intermediate images of the crossover produced by the condenser lenses have significantly larger diameters than the final spot size, the effect of aberrations on these lenses are relatively small. It is thus the effects of spherical and chromatic aberration as well as the astigmatism of the final condenser lens that are critical in the design and performance of the objective lens of SEM.
(b)Magnetic Field. As a result of electron bombardment, secondary electrons in the SEM are emitted over a wide solid angle. These have energies of only few electron volts, yet they must be able to reach the detector to produce the necessary signal. As a result, the magnetic field at the specimen must be designed so that it will not restrict effective secondary electron collection.
(c)Focal Length. The extent of lens aberrations is dependent upon the focal length. Thus, it is desirable to keep the latter as short as possible in order to help minimize the effects of aberrations.
The final lens usually has externally adjustable apertures. Normally, final apertures on the order of 50–70 mm are used to generate smaller, less electron dense spots for secondary electron generation and imaging. Larger apertures, for example, 200 mm, are used to generate larger spots with greater numbers of electrons. These large spots contain a great deal of energy and may damage fragile specimens. They are used primarily to generate X rays for elemental analysis rather than for imaging purposes.
Specimen Manipulation System
The specimen is normally secured to a metal stub and is grounded to prevent the build up of static high voltage charges when the beam electrons strike the specimen. In order to orient the specimen precisely, relative to the electron beam and electron detectors, all SEMs have controls for rotating and traversing the specimen in x, y, and z directions. It is also possible to tilt the specimen in order to enhance the collection of electrons by a particular detector. These movements have a large effect on magnification, contrast, resolution and depth of field. Some improvement can be made in imaging by reorientation of the specimen.
MICROSCOPY, ELECTRON |
483 |
Interaction Of Electron Beam With Specimen
Three basic possibilities exist as to the nature of the beam– specimen interaction used to generate the image:
1.Some primary electrons, depending on the accelerating voltage, penetrate the solid to depths as much as 10 mm. The electrons scatter randomly throughout the specimen until their energy is dissipated by interaction with atoms of the specimen.
2.Some primary electrons collide with or pass close to the nucleus of an atom of the specimen such that there is a change in the electron’s momentum. This results in electron scatter through a large angle and electron reflection from the specimen. Such elastically reflected primary electrons are known as backscattered electrons.
3.Some primary electrons interact with the host atoms so that as a result of collisions, a cascade of secondary electrons is formed along the penetration path. Secondary electrons have energy ranges of 0–50 eV and are the electrons most commonly used to generate the 3D image. The mean path length of secondary electrons in many materials is 1 nm. Thus, although electrons are generated throughout the region excited by the incident beam, only those electrons that originate <1 nm deep in the sample escape to be detected as secondary. The shallow depth of production of detected secondary electrons makes them very sensitive to topography.
In addition to producing backscattered and secondary electrons, specimen–beam interactions also produce photons, specimen currents, Auger electrons and X rays that are characteristic of the probed specimen. These emanations can be detected by X ray or electron spectroscopy for elemental analysis of the specimen surface. However, it is rarely used for biological specimens.
Signal Versus Noise
The signals generated as a result of the electron beam striking a specimen are used to convey different types of information about the specimen. In the usual SEM imaging mode, signals consist of the secondary electrons generated from the spot struck by the electron beam and noise consists of secondary electrons originating at locations away from where the beam struck the specimen. The image quality is eventually expressed by the signal-to-noise (S/N) ratio. In a poor quality image the signal to noise ratio is low. One may achieve a better image by either reducing the noise or raising the signal. Since it is more difficult to reduce the noise level, the signal is usually raised by increasing the electron emissions from the gun. Several methods to accomplish increased electron emissions include: altering the bias settings, decreasing the distance between the anode and the filament, decreasing the distance between the filament and the shield aperture, and using either a lanthanum hexaboride filament or a cold-field emissions gun. A second method to increase the signal is to use slower scan rates on the
484 MICROSCOPY, ELECTRON
specimen. Longer dwell times of the beam on the specimen will generate more secondary electrons from the spot where the beam strikes the specimen. This increase in current, however, carries with it an increased risk of damage to sensitive specimens (9).
Secondary Electron Detection
To collect the secondary electrons, a suitable electrode is held at a positive potential and serves to attract them and produce an emission current. The strength of this signal is proportional to the number of electrons striking the collector. This signal is used, after amplification; to modulate the intensity of the cathode-ray tube (CRT) beam as it moves across the tube face, synchronously with the path of the electron probe across the specimen surface.
Typically, the secondary electron collector is based on the original 1960 scintillator-photomultiplier design of Everhart and Thoronley. In this system, the secondary electrons are accelerated towards the scintillator by a potential difference of a few hundred to a few thousands volts. Upon hitting the scintillator, each electron produces many photons that are guided by the light pipe to the photomultiplier. Each photoelectron triggers a release of two or more secondary electrons at the first electrode (dynode) and process cascades, yielding from 100,000 to 50 million additional electrons. Thus, the photomultiplier reconverts the light to an electron current and provides a high degree of amplification that can be controlled by variation of the voltage applied to the dynodes (8).
Image Recording System
The final magnified image in the SEM is formed on a CRT or monitor. Unlike TEM, in which the electrons interact directly with the photographic medium, SEM images are most often photographed directly from the monitor through the lens of either a 35 mm roll film camera or a larger 4 in. 5 in. (10.6 cm 12.7 cm) sheet film camera. The camera shutter remains open as the electron beam slowly scans across the specimen. A valuable addition to most SEMs is the automatic data display that permits the generation of informational data on the viewing and recording monitors. With this accessory, experiment numbers, dates, accelerating voltages and magnifications may be displayed.
DIAGOSTIC ELECTRON MICROSCOPY
Electron microscopy excels as a diagnostic tool with respect to the detection and identification of both abnormal tissue anatomy and the pathogens responsible for the disease. The value of electron microscopy in difficult diagnostic situations has been demonstrated repeatedly, particularly when there is close coordination between the pathologist and the attending clinician. Ultrastructural study may be applied to a variety of substances including biological materials. By examination of specially prepared tissue sections, changes not perceived by light microscopy can be identified, leading to improved diagnostic interpretations. For example, in certain kidney diseases, such as nephrotic syndrome and Nil disease, the correct diagnosis can be made only by these means, and this in turn affects the
selection of therapy. Similarly, certain neoplasms can be identified definitively only through ultrstructural studies, with obvious implications for treatment and prognosis. Another area of growing importance is the identification of viral particles in biological material. In some instances, ultrastructural study is the only way to establish the presence of a viral infection, and in other instances a diagnosis may be made earlier than by serological methods. The costs of diagnostic electron microscopy are relatively small in light of the benefits to patient care. The following are examples highlighting the use of electron microscopy in the diagnosis of certain diseases.
Neoplasms
Many neoplasms appear undifferentiated by light microscopy, but most show differentiation along one cell line or another at the ultrastructural level. However, it is noteworthy that not all of the ultrastructureal criteria for identifying the cell type may be present in every neoplasm. As expected, the more differentiated the neoplasm, the more likely will its cells contain a broad complement of diagnostic morphologic features. Usually, the ultrastructural findings do allow the pathologist to make a definitive diagnosis when interpreted in conjunction with the light microscopic picture, and in some cases with the histochemical and immunohistochemical results. The example that follows will highlight the use of diagnostic electron microscopy in the identification of carcinomas. Various types of carcinomas have a number of distinguishing features, but one common characteristic of all carcinomas is the presence of intracellular junctions, usually desmosomes and/or intermediate junctions. The presence of lumens, microvilli, tight junctions, junctional complexes, basal lamina, secretory granules, prominent Golgi apparatus, and moderately prominent rough endoplasmic reticulum are all suggestive of adenocarcinoma in the differential diagnosis of a neoplasm (10). Figure 6 shows a pancreatic carcinoma, which may arise from acinar cells, centrocinar cells, intercalated duct cells, interlobular duct cells, interlobular duct cells and main pancreatic duct cells. Adenocarcinomas arising from main and interlobular ducts (mucinous cystadenocarcinomas) have cells similar to those of bile ducts and intestinal epithelium; that is, the cytoplasm contains mucin granules, and the free surface has microvilli filled with thin filaments that anchor into the subjacent cytoplasm (11–13).
Infectious Diseases
Bacteria. Diagnostic criteria for bacterial rods and cocci are (1) the presence of an outer-cell wall, (2) the presence flagella or pili (fimbria) on the outer surface of the cell, (3) the presence of an inner-cell membrane, (4) a central nuclear region (nucleoid), without a limiting membrane, (5) dense cytoplasm composed mostly of ribosomes, and (6) a varying number of vesicles formed from the inner-cell membrane (mesosomes), storage vacuoles and endospores (14). Figure 7 shows the bacteria in Whipple disease. The rods are present both free and within macrophages.
Viruses. The distinct morphology of members of different viral families usually allows an agent to be assigned to
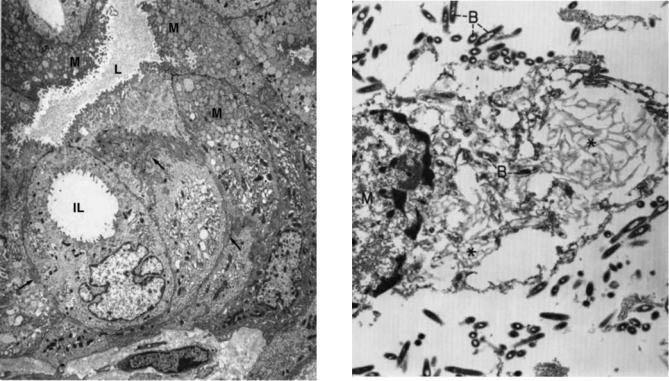
MICROSCOPY, ELECTRON |
485 |
Figure 6. Ductal, mucinous cystadenocarcinoma (pancreas). In this field, the neoplastic cells form a cystic lumen (L) lined by innumerable microvilli. An intracytoplasmic lumen (IL), without junctional complexes, is present in one cell. Some of the cells lining the lumen have a rich collection of mucinous granules (M) in their apical cytoplasm. Lateral cell borders show a switch-backing pattern of interdigitation (arrows). (6800 ) (From Ref. 10. Reproduced by courtesy of Springer-Verlag GmbH.)
a particular family. This morpho-diagnosis, combined with clinical information is often sufficient to permit a provisional diagnosis and to initiate treatment and containment protocols while waiting for other test results. Diagnostic criteria that apply to viruses in general are (1) the presence of intracellular and/or extracellular elliptical, stand-like, round or polygonal structures measuring 20–300 nm in diameter; and (2) the identification of viral morphology, consisting of a central, electron dense core (DNA-containing nucleoid) and an outer shell (capsid), which may have more than one layer (Fig. 8) (15).
Fungi. The electron microscopic diagnostic criteria for fungi include the identification of mononucleated oval yeast forms measuring 2–4 mm in diameter, with a thin cell wall and no true capsule. These can be located either extracellularly or intracellularly (16). A representative fungus, Histoplasma capsulatum, is shown in Fig. 9. The organisms have a clear halo between their visible cytoplasm and their thin cell wall.
Skeletal Muscle Diseases
The skeletal muscle responses to injury that are visible with the electron microscope can be categorized as follows: (1) alterations in the sarcolemma (e.g., discontinuities of
Figure 7. Whippl’s disease. The lamina propria of the jejunal mucosa contained numerous Whipple’s type macrophages [M¼ macrophage nucleus) and bacteria rods (B)]. Most of the intact bacilli are extracellular, whereas those in the macrophage are in various stages of degeneration, including the end-stage of serpiginous membrane ( ). (16,500 ) (From Ref. 10. Reproduced by courtesy of Springer-Verlag GmbH.)
the plasma membrane or the basement membrane); (2) alterations in myofilaments; (3) Z-band alterations (e.g., streaming and nemaline bodies); (4) nuclear changes (e.g., abnormal location of the nucleus within the muscle fiber and nuclear inclusions); (5) abnormalities of the sarcoplasmic reticulum and the T-system (e.g., tubular aggregates), (6) abnormal accumulations of metabolites (e.g., glycogen and lipids); (7) abnormal cytoplasmic structures (e.g., vacuoles, cytoplasmic bodies, concentric laminated bodies, fingerprint bodies, curvilinear bodies). In general, many of these ultrastructural abnormalities are not specific for a single disease. Electron microscopy can be a valuable adjunct to help the pathologist arrive at the proper interpretation of a muscle biopsy when taken together with all other available clinical, electrophysiologic, and histopathological data. In addition to the pathologic changes that might involve the muscle fibers themselves, many diseases of muscle also simultaneously affect adjoining connective tissue components, blood vessels and intramuscular nerves. It is therefore important to pay particular attention to these structures when examining muscle with the light and electron microscope (10). The light micrograph shown in Fig. 10 demonstrates centrally placed nuclei in the majority of the muscle fibers. The central nuclei often are surrounded by a clear area that is devoid of adenosine triphosphatase (ATPase) activity. Ultrastructural features of the paranuclear clear zone in Fig. 11 include (1) the
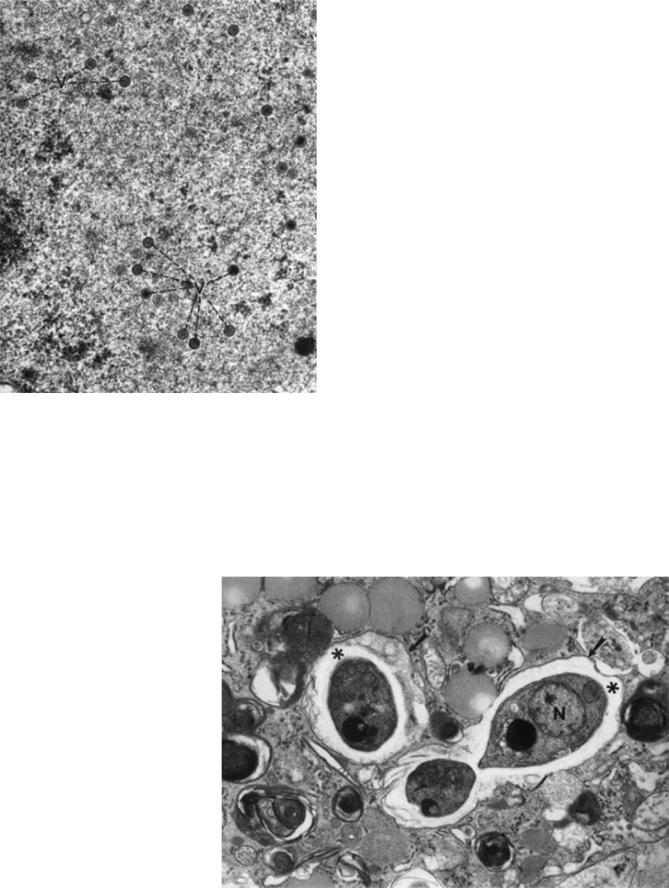
486 MICROSCOPY, ELECTRON
Figure 8. Herpes simplex encephalitis (cerebrum). Virions (V) have a central dense nucleoid and an outer three-layered capsid. (63,800 ) (From Ref. 10. Reproduced by courtesy of SpringerVerlag GmbH.)
absence of myofilaments, (2) numerous mitochondria, (3) glycogen accumulation. In some patients, especially infants, the central clear zone may be more evident than the nuclei within that zone when examining the tissue in cross-section (10).
Figure 9. Histoplasma capsulatum (supraclavicular lymph node). High magnification of parasitic yeast forms illustrates details of their internal structure. N ¼ nucleus; * ¼ clear, peripheral, cytoplasmic halo; arrows ¼ parasitic cell membrane. (20,000 ) (From Ref. 10. Reproduced by courtesy of Springer-Verlag GmbH.)
Peripheral Nerve Diseases
Wallerian Degeneration. Ultrastructural changes detected in peripheral nerve specimens include either the general pathologic responses of the peripheral nerve to either the injury or the specific disease entity that afflicts the patient. The general pathologic processes involving peripheral nerve can be divided into two broad categories: those that indicate a process primarily affecting the axon and those that indicate a process primarily affecting the myelin sheath. Examination of peripheral nerve biopsies by electron microscopy therefore must include evaluation of the axons, the interstitium, and the myelin and Schwann cells.
The sequence of structural changes following nerve injury that are collectively called Wallerian degeneration is shown in Fig. 12. Wallerian degeneration specifically refers to degeneration of the distal segments of a peripheral nerve after severance of the axons from their cell bodies (17). When the nerve injury is a contusion, the basement membrane of the Schwann cell is preserved, allowing regeneration within the endoneurial tube. In contrast, when the nerve injury is a transection, the endoneurial tube (composed of denervated Schawnn cells and extracellular matrix) may not be appropriately aligned with the regenerating axons. Axonal regeneration is therefore less efficient after nerve degeneration that follows a transection injury compared to that following a crush injury (10,17).
PROSPECTS OF ELECTRON MICROSCOPY
In the 1980s, electron microscopy lost much of its former role in the life sciences due to the introduction of modern, highly effective molecular analytical techniques, such as immunohistochemistry, chip technology, and confocal laser scan microscopy. In recent years, however, substantial technical improvements were made in specimen preparation, instrumentation and software, allowing the
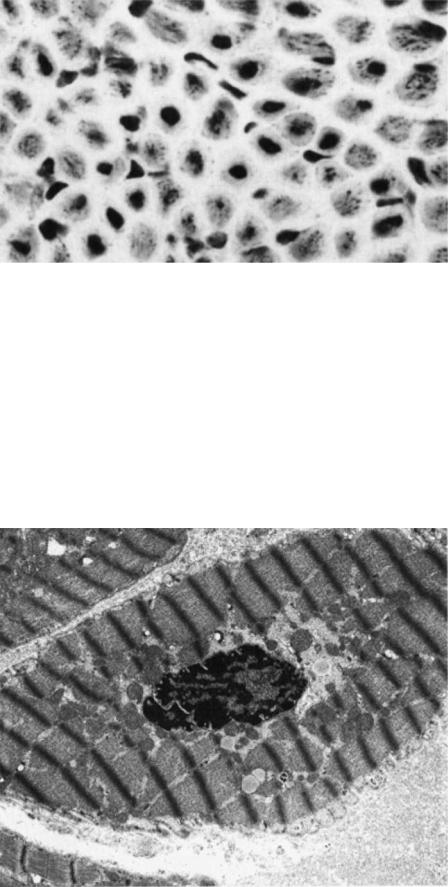
MICROSCOPY, ELECTRON |
487 |
electron microscope to reemerge as a valuable tool for analyzing molecular complexes.
Electron microscopy as an imaging technique allows a direct view of biological objects, while some of the other available techniques are indirect and in some instances nonspecific. Using electron microscopy, all components of the object and their mutual relationships at the molecular level can be analyzed. This information provides insight toward an understanding of structure–function relations.
The possibility of 3D reconstruction of cellular components via electron microscopy, along with the ease and speed with which newer instruments can provide data, have given the way to what many in the field are referring as a revolution. Recent technological advancements
Figure 10. Centronuclear (myotubular) myopathy. The majority of small fibers contain centrally placed nuclei. (H&E, 150 ) (From Ref. 10. Reproduced by courtesy of Springer-Verlag GmbH.)
have made automated data acquisition possible, and have thus allowed a reduction of the total electron dose needed to image a specimen. Specimen preparation advances, such as embedding biological specimens in vitreous ice, have enabled studies of the macromolecular organization of cells. Whole prokaryotic and small eukaryotic cells can be directly grown and hydrated frozen on electron microscopy grids. Examination of the naturally preserved cells delivers images of the cellular structures in their functional environment. Such so-called tomograms contain all available information about the spatial relationships of macromolecular structures within the cell. However, due to their poor S/N and the generally highly crowded nature of the cytoplasm, the interpretation of
Figure 11. Muscle fiber with degenerated nucleus. Note absence of myofilaments and accumulation of glycogen in the paranuclear region to the right (15,000 ). (From Ref. 10. Reproduced by courtesy of SpringerVerlag GmbH.)