
Biomolecular Sensing Processing and Analysis - Rashid Bashir and Steve Wereley
.pdf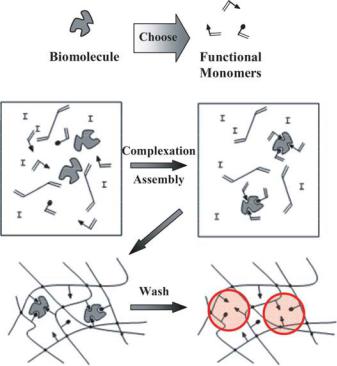
INTELLIGENT POLYMERIC NETWORKS IN BIOMOLECULAR SENSING |
125 |
FIGURE 6.6. Schematic of the biomimetic approach to molecular imprinting. Recognition sites are highlighted with shaded circles.
respond, in theory, to any desired analyte [11]. A schematic of the biomimetic molecular imprinting methodology is included as Figure 6.6.
There are some significant characteristics to consider in the design of biomimetic polymer networks via a molecular imprinting technique. To achieve a relatively easy on/off binding event, a non-covalent recognition process is favored. Therefore, supramolecular interactions, such as hydrogen bonding, electrostatic interactions, hydrophobic interactions, and van der Waals forces, are employed to achieve recognition. For the formation of the network, it is imperative that the functional monomers, crosslinker, and template are mutually soluble. In addition, the solvent must be chosen wisely, so that it does not interact and destabilize the self-assembled functional monomer and template.
6.1.7. Star Polymer Hydrogels
Star polymers are structurally intriguing materials that have been synthesized and characterized in the past fifteen years. Star polymers are hyperbranched polymers with large number of arms emanating from a central core, as illustrated in Figure 6.7. Starshaped polymers are usually prepared by anionic or cationic polymerizations, which provide a relatively compact structure in the form of star-shaped polymers of nanometers size. In recent years, researchers have applied star polymers and other dendritic structures in a variety
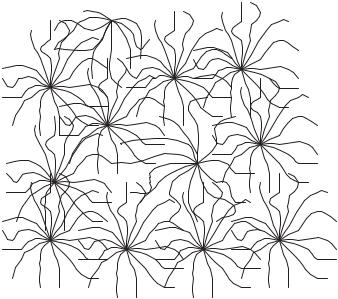
126 |
NICHOLAS A. PEPPAS AND J. ZACHARY HILT |
FIGURE 6.7. Structure of a Star Polymer Gel.
of biomedical and pharmaceutical applications, including tissue engineering, gene delivery, and artificial recognition elements [33–37]. The advantage of star polymers in creating responsive and recognitive networks is the presence of a large number of functional groups in a small volume.
In our lab, we have investigated the use of star polymers as advanced materials for pH-sensitive and recognitive polymer networks [37]. By imprinting star polymer building blocks with a D-glucose template, we demonstrated the ability to synthesize novel polymeric networks that were able to distinguish between the template and a similar sugar, D-fructose. In addition, star polymers were copolymerized with ionizable methacrylic acid to synthesize polymer networks that exhibited sensitivity to changes in the pH. These networks, which are hydrophilic leading to applicability in aqueous environments, are promising as sensing elements for use in biological environments.
6.2. APPLICATIONS OF INTELLIGENT POLYMER NETWORKS AS RECOGNITION ELEMENTS
A number of research groups have utilized hydrogels as functional components in biomedical applications, such as in biomaterials and biosensors. Ito and collaborators [38– 40] have patterned pH-sensitive and thermo-sensitive hydrogels and proposed these microstructures for use in various microdevices. In numerous studies done by Matsuda and coworkers [41–45], hydrogels have been micropatterned using a surface polymerization technique induced by an iniferter (acts as an initiator, a transfer agent, and a terminator) to create surface regions with different physicochemical properties for direction of cell
INTELLIGENT POLYMERIC NETWORKS IN BIOMOLECULAR SENSING |
127 |
adhesion and behavior. In our own laboratory, we have patterned poly(ethylene glycol)- containing hydrogels onto polymer substrates that had incorporated iniferters in their networks to provide bonding to create novel surfaces for possible application in biosensors and in biomaterials for selective adhesion of cells and proteins [46].
Several other groups have focused on developing microdevices utilizing the mechanical response of environmentally sensitive hydrogels for microactuation. Beebe et al. [47, 48] and Zhao et al. [49] have micropatterned pH-sensitive hydrogels inside microfluidic channels to create flow controls that sense the environmental conditions and then actuate in response. In similar work, pH-sensitive hydrogels were patterned to form a biomimetic valve capable of directional flow control [50]. Similarly, Madou and coworkers [51] have utilized a blend of redox polymer and hydrogel to create an “artificial muscle” that can act as an electroactuated microvalve for possible application in controlled drug delivery.
Of particular interest, environmentally sensitive hydrogels have been applied as sensing elements for development of novel sensor platforms, utilizing various transducing elements. Specifically, hydrogels have been applied as recognition matrices where the hydrogel provided stability for an entrapped biological component and/or its properties were monitored with changing environmental conditions. In addition, hydrogels were utilized as actuation elements where the mechanical response of the network was applied to actuate various transduction elements.
6.2.1. Sensor Applications: Intelligent Polymer Networks as Recognition Matrices
Several research groups have patterned hydrogels containing immobilized oxidoreductase enzymes, such as glucose oxidase, lactate oxidase, and alcohol oxidase, onto electrodes using photolithography to create biosensors for monitoring various analyte levels [52–55]. For example, Jimenez et al. [55] fabricated enzymatic microsensors that employed polyacrylamide as a entrapment matrix for immobilization of enzyme recognition elements, including glucose oxidase and urease. To create biosensors selective for glucose or urea, the polymer matrix containing the desired enzyme was patterned onto a pH-sensitive ion selective field-effect transistor (FET). In similar work, Sevilla et al. [56] fabricated a SO2 sensor by coating a pH-sensitive FET with a polyurethane-based hydrogel containing a hydrogen sulfite electrolyte, not an enzyme, entrapped within its network structure. A pH change as a result of the interaction of the sulfur dioxide with the hydrogel matrix was successfully measured.
In other work, Sheppard Jr. et al. [57–59] developed miniature conductimetric pH sensors based on the measurement of the conductivity of pH-sensitive hydrogels that were photo-lithographically patterned onto planar interdigitated electrode arrays [57–59]. The sensor detection was based on the measurement of changes in the electrical conductivity of the hydrogel membrane that resulted with its swelling/collapsing. In related work, Guiseppi-Elie et al. [60] demonstrated chemical and biological sensors that applied conducting electroactive hydrogel composites as recognition elements and utilized electrochemical detection.
In addition, various optical based detection schemes have utilized to hydrogel systems. Leblanc et al. [61] have synthesized hydrogel membranes with a short peptide sequence immobilized within the network as a membrane fluorescent sensor. In this work, the peptide sequence was chosen due to its high binding affinity for Cu2+ and contained
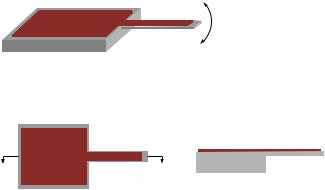
128 |
NICHOLAS A. PEPPAS AND J. ZACHARY HILT |
a dansyl fluorophore for signal transduction. It was demonstrated that the gel film exhibited a fluorescent emission that was selectively and reversibly quenched by copper ions in its aqueous environment. In work by another researcher, pH-sensitive hydrogels were photolithographically patterned for possible application in a fluorescence sensor array [62]. Arregui et al. [63] integrated neutral hydrogels with optical fibers to demonstrate an optical based humidity sensor. Pishko et al. [64] have encapsulated living cells within hydrogel microstructures and demonstrated finite viability of the cells. These systems have possible application as optical biosensor arrays of individually addressable cell-containing hydrogels for drug screening or pathogen detection.
6.2.2. Sensor Applications: Intelligent Polymer Networks as Actuation Elements
Utilizing the actuation response of hydrogels, Grimes et al. [65,66] demonstrated wireless pH sensors based on integrating pH-responsive hydrogels with magnetoelastic thick films. The sensor device functioned by monitoring change in resonance frequency due to applied mass load of the magnetoelastic sensor device by remote query. Recently, Han et al. [67] demonstrated a constant-volume hydrogel osmometer as a novel sensor platform. The concept was illustrated with a device where a pH-responsive hydrogel was confined between a rigid semipermeable membrane and the diaphragm of a miniature pressure sensor. Changes in the osmotic swelling pressure of the hydrogel resulting from changes pH were accurately measured via the pressure sensor. Although the device was of macroscale in dimensions, the design can be easily miniaturized for microscale sensor development. Other groups have demonstrated sensor platforms at the macroscale for pH [68] and CO2 [69] using pressure sensors to transduce the swelling response of hydrogel systems. These systems also have the ability to be miniaturized, which would greatly enhance their applicability.
Recently, microelectromechanical systems (MEMS) sensor platforms, specifically those based on microcantilevers, have been applied in a wide variety of applications due their miniature size and ultrahigh sensitivity. In our work, environmentally responsive hydrogels have been integrated with silicon microcantilevers to develop an ultrasensitive bioMEMS sensor platform (see Figure 6.8). Specifically, a pH microsensor was demonstrated based on
Deflection actuated by the swelling/deswelling of the responsive polymer network
Top View
Side View
FIGURE 6.8. Schematic of MEMS sensor platform based on microcantilever patterned with an intelligent polymer network.
INTELLIGENT POLYMERIC NETWORKS IN BIOMOLECULAR SENSING |
129 |
a methacrylic acid based pH-responsive hydrogel [70, 71]. This was the first demonstration of a microscale MEMS sensor device where actuation is controlled by an intelligent polymer network. In similar work, Thundat et al. [72] have recently demonstrated a variation on our novel sensor platform by integrating hydrogels responsive to CrO42− with commercial silicon microcantilevers to create CrO42− sensors. More recently, another variation has been demonstrated where hydrogels containing benzo-18-crown-6 coated on microcantilevers to create Pb2+ sensors [73].
6.3. CONCLUSIONS
Intelligent polymer networks are exceptional materials for application as novel sensing elements, since the response of the macromolecular network can be precisely tailored through the molecular design of the polymer network functionality and three dimensional structure. These intelligent networks have numerous advantages over natural bioreceptors, such as antibodies and enzymes, due to their low price and robustness. In particular, biomimetic networks can be advantageous over their biological counterparts because they can be designed to mimic biological recognition pathways.
Previously, sensor platforms have been demonstrated using intelligent polymer networks as sensing elements and a variety of transducing elements, such as gravimetric, optical, and electrochemical, but these have mostly been at the macroscale. The further development of microand nanoscale sensors that utilize intelligent polymer networks as functional components, such as those based on microcantilevers, will drastically enhance the diagnostic capabilities in a variety of fields. For instance, the field of clinical diagnostics presents numerous opportunities where microand nanoscale biosensor technology can be exploited by developing lab-on-a-chip and other miniature point-of-care devices that enhance the speed and precision with which health care is administered. The future of biomolecular sensing will be profoundly impacted by the increased integration of intelligent polymer materials as recognition elements, particularly in microand nanoscale sensors.
REFERENCES
[1]L. Clark, Jr., and C. Lions. Electrode systems for continuous monitoring in cardiovascular surgery. Ann. N.Y. Acad. Sci., 102:29, 1962.
[2]C.K. O’Sullivan and C.G. Guilbault. Commercial quartz crystal microbalances–theory and applications.
Biosens. Bioelectron., 14:663, 1999.
[3]B.A. Cavic, G.L. Hayward, and M. Thompson. Acoustic waves and the study of biochemical macromolecules and cells at the sensor-liquid interface. Analyst, 124:1405, 1999.
[4]E. Benes, M. Groschl, W. Burger, and M. Schmid. Sensors based on piezoelectric resonators. Sensor. Actuat. A—Phys., 48:1, 1995.
[5]M. Sepaniak, P. Datskos, N. Lavrik, and C. Tipple. Microcantilever transducers: A new approach to sensor technology. Anal. Chem., 74:568A, 2002.
[6]O. Wolfbeis. Fiber-optic chemical sensors and biosensors. Anal. Chem., 74:2663, 2002.
[7]J. Homola, S.S. Yee, and G. Gauglitz. Surface plasmon resonance sensors: Review. Sensor. Actuat. B—Chem., 54:3, 1999.
[8]E. Bakker and M. Telting-Diaz. Electrochemical sensors. Anal. Chem., 74:2781, 2002.
130 |
NICHOLAS A. PEPPAS AND J. ZACHARY HILT |
[9]S. Subrahmanyam, S. Piletsky, and A. Turner. Application of natural receptors in sensors and assays. Anal. Chem., 74:3942, 2002.
[10]M. Byfield and R. Abuknesha. Biochemical aspects of biosensors. Biosens. Bioelectron., 9:373, 1994.
[11]M.E. Byrne, K. Park, and N.A. Peppas. Molecular imprinting within hydrogels. Adv. Drug Deliver. Rev., 54:149, 2002.
[12]J.Z. Hilt, M.E. Byrne, and N.A. Peppas. Configurational biomimesis in drug delivery: Molecular imprinting of biologically significant molecules. Adv. Drug Deliver. Rev., 11:1599, 2004.
[13]R. McGlennen. Miniaturization technologies for molecular diagnostics. Clin. Chem., 47:393, 2001.
[14]T. Vo-Dinh and B. Cullum. Biosensors and biochips: Advances in biological and medical diagnostics. Fresen. J. Anal. Chem., 366:540, 2000.
[15]A. Manz, N. Graber, and H. Widmer. Miniaturized total chemical-analysis systemsa—novel concept for chemical sensing. Sensor. Actuat. B—Chem., 1:244, 1990.
[16]A. Tudos, G. Besselink, and R. Schasfoort. Trends in miniaturized total analysis systems for point-of-care testing in clinical chemistry. Lab on a Chip, 1:83, 2001.
[17]Y. Liu, C. Garcia, and C. Henry. Recent progress in the development of mu TAS for clinical analysis. Analyst, 128:1002, 2003.
[18]N.A. Peppas. Hydrogels in Medicine and Pharmacy. CRC Press, Boca Raton, FL, 1986.
[19]R. Langer and N.A. Peppas. Advances in biomaterials, drug delivery, and bionanotechnology. AIChE J., 49:2990, 2003.
[20]N.A. Peppas, Y. Huang, M. Torres-Lugo, J.H. Ward, and J. Zhang. Physicochemical, foundations and structural design of hydrogels in medicine and biology. Ann. Revs. Biomed. Eng., 2:9, 2000.
[21]A.M. Lowman and N.A. Peppas. Pulsatile drug delivery based on a complexation/decomplexation mechanism. In S.M. Dinh, J.D. DeNuzzio, and A.R. Comfort, (eds.), Intelligent Materials for Controlled Release, ACS Symposium Series, ACS, Washington, DC, Vol. 728, pp. 30–42, 1999.
[22]N.A. Peppas and P. Colombo. Analysis of drug release behavior from swellable polymer carriers using the dimensionality index. J. Control. Rel., 45:35, 1997.
[23]A.R. Khare and N.A. Peppas. Release behavior of bioactive agents from pH-sensitive hydrogels. J. Biomat. Sci., Polym. Ed., 4:275, 1993.
[24]L. Brannon-Peppas and N.A. Peppas. Equilibrium swelling behavior of pH-sensitive hydrogels. Chem. Eng. Sci., 46:715, 1991.
[25]Y. Qiu and K. Park. Environment-sensitive hydrogels for drug delivery. Adv. Drug Deliv. Revs., 53:321, 2001.
[26]B. Jeong, S.W. Kim, and Y.H. Bae. Thermosensitive sol-gel reversible hydrogels. Adv. Drug Deliv. Revs., 54:37, 2002.
[27]T. Miyata, T. Uragami, and K. Nakamae. Biomolecule-sensitive hydrogels. Adv. Drug Deliv. Revs., 54:79, 2002.
[28]A.M. Lowman and N.A. Peppas. Analysis of the complexation/decomplexation phenomena in graft copolymer networks. Macromolecules, 30:4959, 1997.
[29]N.A. Peppas, P. Bures, W. Leobandung, and H. Ichikawa. Hydrogels in pharmaceutical formulations. Eur. J. Pharm. Biopharm., 50:27, 2000.
[30]R.A. Scott and N.A. Peppas. Compositional effects on network structure of highly cross-linked copolymers of PEG-containing multiacrylates with-acrylic acid. Macromolecules, 32:6139, 1999.
[31]K. Podual, F.J. Doyle III, and N.A. Peppas. Glucose-sensitivity of glucose oxidase-containing cationic copolymer hydrogels having poly(ethylene glycol) grafts. J. Control Rel., 67:9, 2000.
[32]A.J. Lee and K. Park. Synthesis and characterization of sol-gel phase-reversible hydrogels sensitive to glucose. J. Mol. Recognit., 9:549, 1996.
[33]C. Gao and D. Yan. Hyperbranched polymers: From synthesis to applications. Progress in Polymer Science, 29:183, 2004.
[34]J. Jansen, E. van den Berg, and E.W. Meijer. Encapsulation of guest molecules into a dendritic box. Science, 266:1226, 1994.
[35]L.G. Griffith and S. Lopina. Microdistribution of substratum-bound ligands affects cell function: Hepatocyte spreading on PEO-tethered galactose. Biomaterials, 19:979, 1998.
[36]S.C. Zimmerman, M.S. Wendland, N.A. Rakow, I. Zharov, and K.S. Suslick. Synthetic hosts by monomolecular imprinting inside dendrimers. Nature, 418:399, 2002.
INTELLIGENT POLYMERIC NETWORKS IN BIOMOLECULAR SENSING |
131 |
[37]E. Oral and N.A. Peppas. Responsive and recognitive hydrogels using star polymers. J. Biomed. Mater. Res., 68A:439, 2004.
[38]Y. Ito. Photolithographic synthesis of intelligent microgels. J. Intell. Mater. Syst. Struct. 10:541, 1999.
[39]G. Chen, Y. Imanishi, and Y. Ito. Photolithographic synthesis of hydrogels. Macromolecules, 31:4379, 1998.
[40]G. Chen, Y. Ito, and Y. Imanishi. Micropattern immobilization of a pH-sensitive polymer. Macromolecules, 30:7001, 1997.
[41]Y. Nakayama, J.M. Anderson, and T. Matsuda. Laboratory-scale mass production of a multi-micropatterned grafted surface with different polymer regions. J. Biomed. Mater. Res. (Appl. Biomater.), 53:584, 2000.
[42]K.M. DeFife, E. Colton, Y. Nakayama, T. Matsuda, and J.M. Anderson. Spatial regulation and surface chemistry control of monocyte/macrophage adhesion and foreign body giant cell formation by photochemically micropatterned surfaces. J. Biomed. Mater. Res., 45:148, 1999.
[43]J. Higashi, Y. Nakayama, R.E. Marchant, and T. Matsuda. High-spatioresolved microarchitectural surface prepared by photograft copolymerization using dithiocarbamate: Surface preparation and cellular responses. Langmuir, 15:2080, 1999.
[44]Y. Nakayama, K. Nakamata, Y. Hirano, K. Goto, and T. Matsuda. Surface hydrogelation of thiolated watersoluble copolymers on gold. Langmuir, 14:3909, 1998.
[45]Y. Nakayama and T. Matsuda. Surface macromolecular architectural designs using photo-graft copolymerization based on photochemistry of benzyl N,N-diethyldithiocarbamate. Macromolecules, 29:8622, 1996.
[46]J.H. Ward, R. Bashir, and N.A. Peppas. Micropatterning of biomedical polymer surfaces by novel UV polymerization techniques. J. Biomed. Mater. Res., 56:351, 2001.
[47]D.J. Beebe, J.S. Moore, J.M. Bauer, Q. Yu, R.H. Liu, C. Devadoss, and B. Jo. Functional hydrogel structures for autonomous flow control inside microfluidic channels. Nature, 404:588, 2000.
[48]D.J. Beebe, J.S. Moore, Q. Yu, R.H. Liu, M.L. Kraft, B. Jo, and C. Devadoss. Microfluidic tectonics: A comprehensive construction platform for microfluidic systems. Proc. Natl. Acad. Sci. U.S.A., 97:13488, 2000.
[49]B. Zhao and J.S. Moore. Fast pHand ionic strength-responsive hydrogels in microchannels. Langmuir, 17:4758, 2001.
[50]Q. Yu, J.M. Bauer, J.S. Moore, and D.J. Beebe. Responsive biomimetic hydrogel valve for microfluidics.
Appl. Phys. Lett., 78:2589, 2001.
[51]L. Low, S. Seetharaman, K. He, and M.J. Madou. Microactuators toward microwaves for responsive controlled drug delivery. Sens. Actu. B, 67:149, 2000.
[52]K. Sirkar and M.V. Pishko. Amperometric biosensors based on oxidoreductases immobilized in photopolymerized poly(ethylene glycol) redox polymer hydrogels. Anal. Chem., 70:2888, 1998.
[53]A. Munoz, R. Mas, C.A. Galan-Vidal, C. Dominiguez, J. Garcia-Raurich, and S. Alegret. Thin-film microelectrodes for biosensing. Quimica. Analytica. 18:155, 1999.
[54]G. Jobst, I. Moser, M. Varahram, P. Svasek, E. Aschauer, Z. Trajanoski, P. Wach, P. Kotanko, F. Skrabal, and G. Urban. Thin-film microbiosensors for glucose-lactate monitoring. Anal. Chem., 68:3173, 1996.
[55]C. Jimenez, J. Bartrol, N.F. de Rooij, and M. Koudelka. Use of photopolymerizable membranes based on polyacrylamide hydrogels for enzymatic microsensor construction. Anal. Chim. Acta., 351:169, 1997.
[56]B.S. Ebarvia, C.A. Binag, and F. Sevilla III. Surface and potentiometric properties of a SO2 sensor based on a hydrogel coated pH-FET. Sens. Actu. B, 76:644, 2001.
[57]N.F. Sheppard, Jr., M.J. Lesho, P. McNally, and A.S. Francomacaro. Microfabricated conductimetric pH sensor. Sens. Actu. B, 28:95, 1995.
[58]M.J. Lesho and N.F. Sheppard, Jr., Adhesion of polymer films to oxidized silicon and its effect on performance of a conductometric pH sensor. Sens. Actu. B, 37:61, 1996.
[59]N.F. Sheppard Jr., R.C. Tucker, and S. Salehi-Had. Design of a conductimetric pH microsensor based on reversibly swelling hydrogels. Sens. Actu. B, 10:73, 1993.
[60]S. Brahim, A.M. Wilson, D. Narinesingh, E. Iwuoha, and A. Guiseppi-Elie. Chemical and biological sensors based on electrochemical detection using conducting electroactive polymers. Microchim. Acta, 143:123, 2003.
[61]Y. Zheng, K.M. Gattas-Asfura, C. Li, F.M. Andreopoulos, S.M. Pham, and R.M. Leblanc. Design of a membrane fluorescent sensor based on photo-cross-linked PEG hydroge. J. Phys. Chem. B, 107:483, 2003.
[62]A. Revzin, R.J. Russell, V.K. Yadavalli, W. Koh, C. Deister, D.D. Hile, M.B. Mellott, and M.V. Pishko. Fabrication of poly(ethylene glycol) hydrogel microstructures using photolithography. Langmuir, 17:5440, 2001.
132 |
NICHOLAS A. PEPPAS AND J. ZACHARY HILT |
[63]F.J. Arregui, Z. Ciaurriz, M. Oneca, and I.R. Matias. An experimental study about hydrogels for the fabrication of optical fiber humidity sensors. Sens. Actu. B, 96:165, 2003.
[64]K. Koh, A. Revzin, and M.V. Pishko. Poly(ethylene glycol) hydrogel microstructures encapsulating living cells. Langmuir, 18:2459, 2002.
[65]C. Ruan, K.G. Ong, C. Mungle, M. Paulose, N.J. Nickl, and C.A. Grimes. A wireless pH sensor based on the use of salt-independent micro-scale polymer spheres. Sens. Actu. B, 96:61, 2003.
[66]C. Ruan, K. Zeng, and C.A. Grimes. A mass-sensitive pH sensor based on a stimuli-responsive polymer.
Anal. Chim. Acta, 497:123, 2003.
[67]I. Han, M. Han, J. Kim, S. Lew, Y.J. Lee, F. Horkay, and J.J. Magda. Constant-volume hydrogel osmometer: A new device concept for miniature biosensors. Biomacromolecules, 3:1271, 2002.
[68]L. Zhang and W.R. Seitz. A pH sensor based on force generated by pH-dependent polymer swelling. Anal. Bioanal. Chem., 373:555, 2002.
[69]S. Herber, W. Olthuis, and P. Bergveld. A swelling hydrogel-based P-CO2 sensor. Sens. Actu. B, 91:378, 2003.
[70]R. Bashir, J.Z. Hilt, A. Gupta, O. Elibol, and N.A. Peppas. Micromechanical cantilever as an ultrasensitive pH microsensor. Appl. Phys. Lett., 81:3091, 2002.
[71]J.Z. Hilt, A.K. Gupta, R. Bashir, and N.A. Peppas. Ultrasensitive biomems sensors based on microcantilevers patterned with environmentally responsive hydrogels. Biomed. Microdev., 5:177, 2003.
[72]Y. Zhang, H. Ji, G.M. Brown, and T. Thundat. Detection of CrO42using a hydrogel swelling microcantilever sensor. Anal. Chem., 75:4773, 2003.
[73]K. Liu and H. Ji. Detection of Pb2+ using a hydrogel swelling microcantilever sensor. Anal. Sci., 20:9, 2004.
II
Processing and Integrated Systems
7
A Multi-Functional Micro Total Analysis System (µTAS) Platform for Transport and Sensing of Biological Fluids using Microchannel Parallel Electrodes
Abraham P. Leea,b, John Collinsa, and Asuncion V. Lemoff c
a Department of Biomedical Engineering
b Department of Mechanical & Aerospace Engineering, University of California at Irvine, 204 Rockwell Engineering Center, Irvine, CA 92697-2715, U.S.A.
c Biotechnology Consultant, Union City, CA 94587, U.S.A.
7.1. INTRODUCTION
The field of micro total analysis systems (µTAS) is developing technologies to integrate sample acquisition, sample separation, target purification, and cellular/molecular level detection schemes on microscale common platforms. The foundation of µTAS is a fluid transport platform that enables the manipulation of small volumes of fluids in microscale channels and chambers. Ideally, it would function much like integrated circuits (IC), except that it would be dealing with fluids instead of electrons. For µTAS, in lieu of the voltage sources would be micropumps and for transistors the microvalves and microfluidic switches. In ICs, batch fabrication processes such as CMOS (complementary metal-oxide semiconductors) have enabled a low cost (per chip), multi-functional design platform that integrates logic and control elements on the same chip. Analogously, the same impact can be made on µTAS if an integrated batch fabrication process is developed for high density complex fluidic routing. However, integrated microfluidics for µTAS faces much tougher