
Biomolecular Sensing Processing and Analysis - Rashid Bashir and Steve Wereley
.pdf186 |
JOEL VOLDMAN |
[82]P. Van Gerwen, W. Laureyn, W. Laureys, G. Huyberechts, M.O. De Beeck, K. Baert, J. Suls, W. Sansen, P. Jacobs, L. Hermans, and R. Mertens. Nanoscaled interdigitated electrode arrays for biochemical sensors.
Sens. Actu. B-Chem., 49:73–80, 1998.
[83]J. Voldman, R.A. Braff, M. Toner, M.L. Gray, and M.A. Schmidt. Holding forces of single-particle dielectrophoretic traps. Biophys. J., 80:531–541, 2001.
[84]J. Voldman, M. Toner, M.L. Gray, and M.A. Schmidt. A microfabrication-based dynamic array cytometer. Anal. Chem., 74:3984–3990, 2002.
[85]J. Voldman, M. Toner, M.L. Gray, and M.A. Schmidt. Design and analysis of extruded quadrupolar dielectrophoretic traps. J. Electrostat. 57:69–90, 2003.
[86]X. Wang, X.-B. Wang, F.F. Becker, and P.R.C. Gascoyne. A theoretical method of electrical field analysis for dielectrophoretic electrode arrays using Green’s theorem. J. Phys. D (Applied Physics), 29:1649–1660, 1996.
[87]X. Wang, X.-B. Wang, and P.R.C. Gascoyne. General expressions for dielectrophoretic force and electrorotational torque derived using the Maxwell stress tensor method. J. Electrostat., 39:277–295, 1997a.
[88]X.-B. Wang, Y. Huang, P.R.C. Gascoyne, and F.F. Becker. Dielectrophoretic manipulation of particles. IEEE Trans. Ind. Appl., 33:660–669, 1997b.
[89]X.-B. Wang, Y. Huang, X. Wang, F.F. Becker, and P.R.C. Gascoyne. Dielectrophoretic manipulation of cells with spiral electrodes. Biophys. J., 72:1887–1899, 1997c.
[90]X. J. Wang, J. Yang, and P.R.C. Gascoyne. Role of peroxide in AC electrical field exposure effects on Friend murine erythroleukemia cells during dielectrophoretic manipulations. Biochimi. Et Biophys. Acta-Gen. Sub., 1426:53–68, 1999.
[91]M. Washizu and T.B. Jones. Multipolar dielectrophoretic force calculation. J. Electrostat., 33:187–198, 1994.
[92]M. Washizu. and T. B. Jones. Generalized multipolar dielectrophoretic force and electrorotational torque calculation. J. Electrostat., 38:199–211, 1996.
[93]J.C. Weaver, T.E. Vaughan, and G.T. Martin. Biological effects due to weak electric and magnetic fields: The temperature variation threshold. Biophys. J., 76:3026–3030, 1999.
[94]J. Wu. Acoustical tweezers. J. Acoust. Soc. Am., 89:2140–2143, 1991.
[95]G. Zhou, M. Imamura, J. Suehiro, and M. Hara. A dielectrophoretic filter for separation and collection of fine particles suspended in liquid. Conference Record of the IEEE Industry Applications Conference, 2:1404–1411, 2002.
[96]X.F. Zhou, J.P.H. Burt, and R. Pethig. Automatic cell electrorotation measurements: Studies of the biological effects of low-frequency magnetic fields and of heat shock. Phys. Med. Biol., 43:1075–1090, 1998.
[97]X.F. Zhou, G.H. Markx, and R. Pethig. Effect of biocide concentration on electrorotation spectra of yeast cells. Biochim. Et Biophys. Acta-Biomem., 1281:60–64, 1996.
[98]U. Zimmermann. Electrical Breakdown, Electropermeabilization and Electrofusion. Rev. Phys. Biochem. Pharma., 105:175–256, 1986.
9
BioMEMS for Cellular
Manipulation and Analysis
Haibo Li, Rafael Gomez´ -Sjoberg¨ a, and Rashid Bashir
Birck Nanotechnology Center and Bindley Biosciences Center, Discovery Park, School of Electrical and Computer Engineering, Purdue University, Weldon School of Biomedical Engineering, Purdue University, West Lafayette, IN. 47907, USA
aNow at Department of Bioengineering, Stanford Univ. Stanford, CA. USA
9.1. INTRODUCTION
Since the introduction of Micro-electro-mechanical systems in the early 70’s, the significance of the biomedical applications of these miniature systems has been realized [54, 77]. BioMEMS, the abbreviation for Biomedical or Biological Micro-Electro-Mechanical- Systems, is now a heavily researched area with a wide variety of important biomedical applications. In general, BioMEMS, and its synonym BioChip, can be defined as “Devices or systems, constructed using techniques inspired from micro/ nanoscale fabrication, that are used for processing, delivery, manipulation, analysis, or construction of biological and chemical entities”. A large number of BioMEMS devices and applications have been presented in [4, 34, 41, 57]. Technologies such as “lab-on-a-chip” and Micro-Total-Analysis-Systems (micro-TAS or µTAS), when used for biological applications, fall into the BioMEMS category. The use of these lab-on-a-chips for cellular analysis is justified by, (i) reducing the sensor element to the scale of size of cells and smaller and hence providing a higher sensitivity, (ii) reduced reagent volumes and associated costs, (iii) reduced time to result due to small volumes resulting in higher effective concentrations, (iv) amenability of portability and miniaturization of the entire system, and (v) ability to perform large numbers of assays or measurements in parallel.
Additionally, technologies that make possible the direct manipulation, probing and detection of individual cells (human, bacterial, from animals and plants) are of great interest
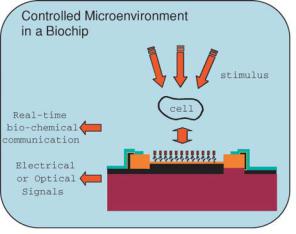
188 |
´ |
¨ |
HAIBO LI, RAFAEL GOMEZ-SJOBERG, AND RASHID BASHIR |
FIGURE 9.1. Micro-fluidic devices with controlled micro-environments for study of cells and the real time profiling of their proteins, mRNA, and other biochemicals (Reprinted with permission from Advanced Drug Delivery Reviews, vol. 56, 2004 and with kind permission from R. Bashir).
to biotechnology researchers and industries. These technologies can greatly facilitate the study of cellular physiology, structure and properties; development and testing of new drugs; rapid detection of pathogenic bacteria; etc. Because of the scales at which biochips operate, they are ideally suited for these applications. For example, until recently many electrical, mechanical and optical characteristics of cells had to be measured as averages over large numbers of cells, and sometimes the effects of extra-cellular materials could not be easily eliminated. Biochips provide an ideal platform for directly measuring electrical, mechanical, and optical properties of individual cells. Electrodes, channels, chambers, etc., in which cells can be manipulated and probed, are readily microfabricated with sizes similar to those of cells ( 1 µm for bacterial cells, 10 µm for mammalian cells).
BioMEMS holds a lot of promise for the analysis of single cells and the study of their function in real time. Micro and nano-scale systems and sensors could allow us to precisely measure the protein, mRNA, and chemical profiles of cells in real time, as a function of controlled stimulus and increase understanding of signaling pathways inside the cell. The development of micro-systems, as schematically shown in Fig. 9.1 [3], where cells can be precisely place, manipulated, lysed, and then analyzed using micro and nano-sensors in ‘real-time’, would have a significant impact on Systems Biology. Integration of sensors for detection of DNA, mRNA, proteins, and other parameters indicating cellular conditions such as oxygen, pH, etc. can be accomplished using BioMEMS platforms and nano-scale sensors. The following subsections will describe some microfabricated devices used for the manipulation, separation and detection of cells.
9.2. BIOMEMS FOR CELLULAR MANIPULATION AND SEPARATION
The ability to manipulate particles, especially living cells, in three-dimensional space is fundamental to many biological and medical applications, including isolation and detection
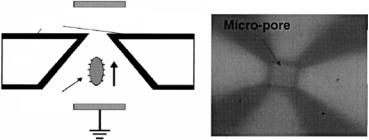
BIOMEMS FOR CELLULAR MANIPULATION AND ANALYSIS |
189 |
of sparse cancer cells, concentration of cells from dilute suspensions, separation of cells according to specific properties, and trapping and positioning of individual cells for characterization. The current methods commonly used for manipulation, concentration and separation of biological particles employ several kinds of physical forces from mechanical, hydrodynamic, ultrasonic, optical, and electro-magnetic origins [73]. Among these methods, electrophoresis and dielectrophoresis (DEP) allow the non-invasive electrical manipulation and characterization of particles, including biological cells, by directly exploiting their electrical and dielectric properties.
9.2.1. Electrophoresis
Electrophoresis is the motion of a charged particle under the influence of an electric field, where the magnitude and direction of the force exerted on the particle are directly proportional to its charge. This effect is most commonly used in biological analysis for separation of DNA and proteins. Most cells have a net charge which will make them move when an electric field is applied. The direction and speed in which the cells move depend on the polarity and magnitude of the charge, respectively, so that cells with different polarities and amounts of charge can be separated by electrophoresis [49]. The electrophoretic mobility of a charged spherical particle can be approximated as:
υ |
= |
q |
(9.1) |
|
|
E6π ηa
where υ is the velocity of the particle, E is the electric field, q is the net charge on the particle, η is the viscosity of the solution and is the radius of the particle.
Poortinga et al. [58] used electrophoresis to deposit bacterial films onto electrodes. Chang et al. [9] used a micro-pore fabricated in an oxide-coated silicon diaphragm and placed between two chambers containing ionic buffer solutions to electrically characterize live and heat-inactivated Listeria innocua bacterial cells as shown in Fig. 9.2. Passage of the electrophoretically-driven cells through the pore was detected by the temporary decrease in the ionic current across the pore caused by the partial blockage of the pore by the traversing
+ ve Voltage
SiO2
Si
Charged
Entity
(a) |
(b) |
FIGURE 9.2. (a) Cross section of the micro-pore device along with the measurement concept, (b) an optical micrograph of the micro-pore, with pore size of 4um on a side (Reprinted with permission from Journal of Vacuum Science & Technology B, vol. 20, no. 5, 2002 and with kind permission from R. Bashir).
190 |
´ |
¨ |
HAIBO LI, RAFAEL GOMEZ-SJOBERG, AND RASHID BASHIR |
cells. Studies of the electrophoretic movement of live and heat-inactivated Listeria innocua cells on interdigitated fingered electrodes demonstrated that live cells have a net negative charge, while heat-inactivated ones are either neutral or positively charged.
9.2.2. Dielectrophoresis
Dielectrophoretic (DEP) forces occur when a non-uniform electric field interacts with a field-induced electrical dipole in a particle. The time-averaged dielectrophoretic force F for a dielectric sphere immersed in a medium is represented as [23, 55, 73]:
F = 2π ε0εm r 3Re[ fCM] |ERMS|2 |
(9.2) |
where ε0 is the vacuum dielectric constant, r is the particle radius, ERMS is the root mean square of the electric field, and fCM is known as the Clausius-Mossotti factor, defined as [23, 73]:
fCM = |
εp − εm |
(9.3) |
||
ε |
+ |
2ε |
||
|
p |
m |
|
where εp and εm are the relative complex permittivities of the particle and the medium respectively. These permittivities depend on the frequency of the applied field. When the permittivity of the particle is larger than that of the medium, Re[ fCM] > 0, the DEP is called positive and the particle moves towards the locations with the highest electric field gradient. When the permittivity of the particle is smaller than that of the medium, Re[FCM] < 0, the DEP is called negative and the particle moves to the locations with the lowest electric field gradient. Early studies of cell dielectrophoresis employed electrode structures made from thin wires and foils, such as cone-plate electrodes in a levitation system [29, 31], simple pin-plate structures [46], and four-pole electrodes for characterization of cell plasma membranes [14]. More recently, MEMS technology has been used for the production of a number of devices with electrode arrays and chambers suitable for manipulation and measurement of biological objects. Integrated dielectrophoresis biochips provide the advantages of speed, flexibility, controllability, and ease of automation [23]. Examples include the fluid-integrated-circuit for single cell handling [74], polynomial electrode arrays for cell separation by trapping cells of different types at different locations using positive and negative DEP forces [25, 43, 72], and various three-dimensional arrays for cell positioning, trapping and levitation [52, 59, 68]. Among all of the above it is in trapping and cell separation that dielectrophoresis has its major applications. Significant examples include separations of viable and nonviable yeast cells [24, 45], leukemia [5] and breast-cancer [6] cells from blood, and the concentration of CD34+ cells from peripheral-stem-cell harvests [61]. Particularly promising is the combination of dielectrophoretic forces with hydrodynamic forces, such as the combined dielectrophoretic-field flow fractionation (DEPFFF) technique for continuous separation [44].
The heart of any dielectrophoresis system is formed by the electrodes from which the driving electric field is applied. The dependence of dielectrophoretic force on both the magnitude and the gradient of the applied electric field means that the electrode configuration needed for efficient dielectrophoretic collection should produce strong, highly non-uniform
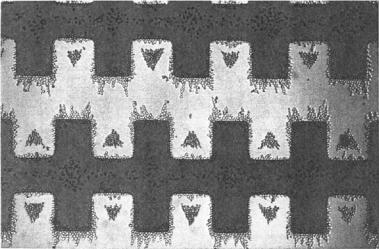
BIOMEMS FOR CELLULAR MANIPULATION AND ANALYSIS |
191 |
FIGURE 9.3. Dielectrophoretic separation of viable and non-viable yeast cells using interdigitated, castellated electrodes. The viable yeast cells collect on the edges of and in pearl chains between the electrodes, whilst the non-viable cells collect in triangular aggregation between the electrodes and in diamond-shaped formations on top of the electrodes (Reprinted with permission from Journal of Biotechnology, vol. 32, 1994 and with kind permission from G. H. Markx).
fields. In this respect microfabricated electrodes present a very clear advantage over macroscale ones because of the ability to create intense and highly non-uniform fields at low voltages (the voltage needed to produce a given field strength is directly proportional to the distance between electrodes). The required electrode configuration can be readily realized using planar micro-fabrication processes with a suitable choice of substrate, usually glass or silicon. The interdigitated electrode array is perhaps the simplest electrode structure that has been used in microbiological applications of dielectrophoresis [73]. From straightforward electrostatic considerations it is clear that the interdigitated electrode configuration has the electric field maximum in both its gradient and strength at the edges of the electrodes and minimum at the centers of electrodes and inter-electrode gaps. Separation of live and heat-treated Listeria bacteria was achieved on the microfabricated interdigitated electrodes reported by Li et al. [38]. Fig. 9.3 shows the separation of viable and non-viable yeast cells using interdigitated, castellated electrodes reported by Markx et al. [45].
A common electrode configuration used for negative dielectrophoresis research is a quadrupole arrangement where four electrodes point towards a central enclosed region [25] as shown in the schematic in Fig. 9.4 (left). By applying an AC field between adjacent electrodes with a phase angle of 180◦ particles will experience dielectrophoresis with a well-defined electric field minimum at the center of the electrode array and maxima at the electrode edges as shown in Fig. 9.4 (right) [26]. If the phase angle is reduced such that adjacent electrodes differ by 90◦, particles will experience both electrorotation and dielectrophoresis and this technique can be used to trap single cells at specific sites.
More advanced and complex electrode structures have been developed for dielectrophoresis studies. Voldman et al. [67, 68, 70, 71] developed a 3-D extruded quadrupole
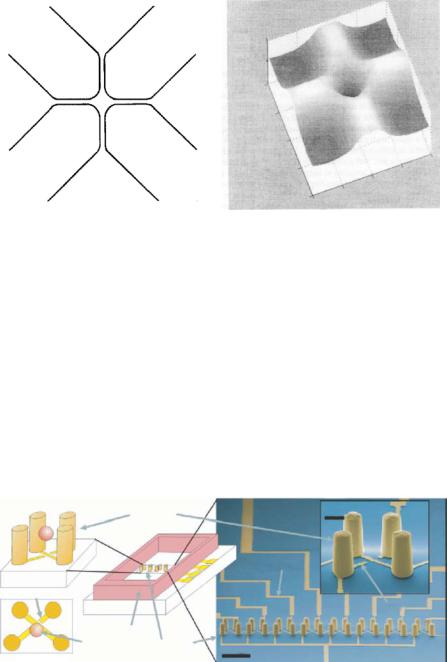
192
a
d
b
c
´ ¨
HAIBO LI, RAFAEL GOMEZ-SJOBERG, AND RASHID BASHIR
Normalised |
electricfield |
1 |
|
|
0.5 |
|
|
||
|
|
0 |
|
|
|
|
-10 |
|
|
|
|
-5 |
|
|
|
|
0 |
|
|
|
|
m |
|
10 |
|
|
5 |
|
5 |
|
|
|
|
|
|
|
|
|
0 |
|
|
10 -10 |
-5 |
m |
|
|
|
FIGURE 9.4. A schematic diagram of typical quadrupole electrode microstructure used in dielectrophoresis experiments (left). The gap between opposing electrodes in the center of the array is typically of the order 10–50 µm across, but can be as small as 500nm or as large as 1mm. The right figure shows a simulation of the electric field in the plane 5 µm above the electrode array shown in the left. The dark region at the center of the electrode array is the electric field minimum, surrounded by a ring of high electric field gradient. Particles experiencing negative dielectrophoresis are repelled into this minimum and become trapped. The electric field strength is high (white region) along the electrode edges, where particles experiencing positive dielectrophoresis will collect (Reprinted with permission from Nanotechnology, vol. 11, 2000 and with kind permission from M.P. Hughes).
structure as shown in Fig. 9.5 [70]. Each of the DEP traps in the array is electrically switchable and capable of holding a single cell, and provides better holding ability than a planar quadrupole trap.
T. Muller¨ et al. [52] designed and constructed several 3-D microelectrode systems consisting of two metal layers with electrode structures acting as funnel, aligner, cage and
(A) |
(B) |
|
(C) |
|
gold posts |
|
20 m |
|
|
|
|
|
|
|
interconnects |
isometric view |
|
|
|
substrate shunt |
|
|
|
glass chip |
|
|
substrate shunt |
SU-8 chamber |
|
|
|
bioparticle |
trap array |
100 |
m |
|
|
top view
FIGURE 9.5. Schematic of the extruded quadrupole DEP trap array. (A) Two views of a single trap, illustrating the trapezoidal placement of the gold posts and a bioparticle suspended in the trap. (B) The trap is one of an array of traps. (C) SEMs of a 1 × 8 array of traps along with an exploded view of one trap (Reprinted with permission from Transducers, 2001 and with kind permission from J. Voldman).
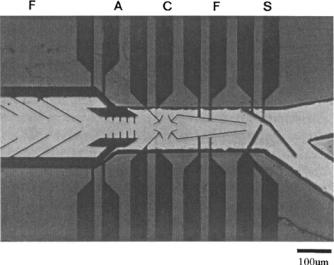
BIOMEMS FOR CELLULAR MANIPULATION AND ANALYSIS |
193 |
FIGURE 9.6. A 3-D microelectrode system. The electrode elements ‘funnel’ (F), ‘aligner’ (A), ‘cage’ (C), and ‘switch’ (S) are arranged over a small distance. Two identical layers of electrodes were overlayed and separated by a 40 µm polymer spacer forming channels (bright region) (Reprinted with permission from Biosensors and Bioelectronics, vol. 14, 1999 and with kind permission from T. Muller)¨ .
switch, separated by a 40µm thick polymer spacer forming a flow channel for handling and caging single cells and particles as shown in Fig. 9.6.
There have been a number of approaches to the application of dielectrophoretic techniques to the ‘lab-on-a-chip’ systems. Some researchers use dielectrophoresis as a method for isolating specific cells at a preliminary stage for further manipulation and/or analyses such as polymerase chain reaction (PCR), electroporation, or cell detection by biochemical methods. Others use dielectrophoresis to perform a range of functions, from separation to trapping and analysis [27]. ‘Lab-on-a-chip’ system for cell separation on microfabricated electrodes using dielectrophoretic/gravitational field-flow fractionation [79].
Recently Li et al. [39, 40] studied a microfabricated dielectrophoretic filter device with a thin chamber and interdigitated electrode array as a test bed for dielectrophoretic trapping of polystyrene beads and biological entities such as yeast cells, spores and bacteria. The device was characterized by both measurement and finite-element modeling of the holding forces against destabilizing flow-induced forces in both positive and negative dielectrophoretic traps. The combination of experiments and modeling has given insight into the DEP forces over the interdigitated electrodes and can be very useful in designing and operating a dielectrophoretic barrier or filter to sort and select biological particles for further analysis.
9.3. BIOMEMS FOR CELLULAR DETECTION
BioMEMS can combine a biologically sensitive element with a physical or chemical transducer to selectively and quantitatively detect the presence of specific compounds in
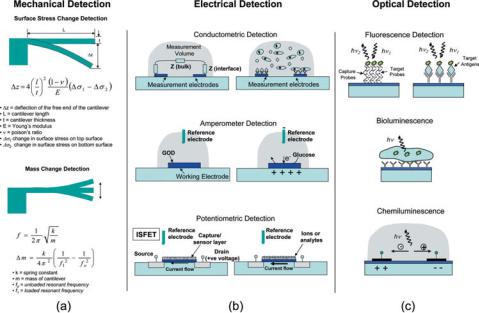
194 |
´ |
¨ |
HAIBO LI, RAFAEL GOMEZ-SJOBERG, AND RASHID BASHIR |
FIGURE 9.7. Some detection modalities used in BioMEMS and Biochip sensors (Reprinted with permission from Advanced Drug Delivery Reviews, vol. 56, 2004 and with kind permission from R. Bashir).
a given environment [67]. During the last decade, BioMEMS devices have been used as biosensors for sensitive, rapid, and real-time measurements [35, 75]. These BioMEMS sensors can be used to detect cells, proteins, DNA, or small molecules. Many demonstrations to date have been of single-sensor devices, but the biggest potential of BioMEMS lies on the ability to create massively parallel arrays of sensors. There are many detection methods used in BioMEMS sensors, including (i) Optical, (ii) Mechanical, (iii) and Electrical. Fig. 9.7 [3] shows a schematic of these key detection modalities as they are used in Biochips and BioMEMS sensors for detection of a wide variety of biological entities. In this review, however, we will focus on detection of cells.
9.3.1. Optical Detection
Optical detection techniques are perhaps the most common and prevalently used in biology and life-sciences. They can be based on fluorescence or chemiluminescence. Fluorescence detection techniques are based on fluorescent markers chemically linked to, for example, DNA or RNA strands or antibodies, which emit light at specific wavelengths in response to an external optical excitation. The presence, enhancement, or reduction in the fluorescence signal can indicate a binding reaction, as shown schematically in Fig. 9.7(c). Recent advances in fluorescence detection technology have enabled single molecule detection [50, 53, 67]. Fluorescence based detection in BioMEMS has been applied to detection of cells within micro-chips, using antibody-based assays (Enzyne-Linked ImmunoSorbent Assay, ELISA, type) as shown in Fig. 9.8 [50, 62].
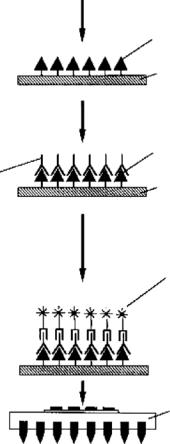
BIOMEMS FOR CELLULAR MANIPULATION AND ANALYSIS |
195 |
Immobilization of E. Coli Cells on
Sample platform
|
Immobilization of E. Coli |
|
Cells |
|
Sample Platform |
|
(Zeta Probe Membrane) |
Exposure to Anti E. Coli Antibodies |
|
|
Immobilization of E. Coli |
|
Cells |
Immobilized |
Sample Platform |
Oost. Anti-E.Coli |
|
Antibodies |
(Zeta Probe Membrane) |
Immobilization of Cy5-Labeled Probe |
|
|
Immobilized Cy5-Labeled |
|
Rabbit Anti-Craft |
|
Antibodies |
Delection
IC Biochip
FIGURE 9.8. Optical detection of E.coli using fluorescently labeled antibodies on a chip (Reprinted with permission from Fresenius Journal of Analytical Chemistry, Vol. 369, 2001 and with kind permission from T. Vo-Dinh).
Chemiluminescence is the light generated by the release of energy as a result of a chemical reaction. Light emission from a living organism is commonly termed bioluminescence (sometimes called biological fluorescence), and light emission which takes place under excitation by an electrical current is designated electrochemiluminescence. One of the challenges for optical detection within biochips is the ability to integrate light sources and detectors in a miniaturized portable format. This integration requires fabrication of photo-diodes in silicon substrates [30] or heterogeneous integration of compound semiconductor LEDs and photodetectors within plastic or polymer platforms [10]. In the later study, microassembly of a hybrid fluorescence detection microsystem was demonstrated by heterogeneous integration of a CdS thin-film filter, an (In,Ga)N thin-film blue LED, and a disposable PDMS microfluidic device onto a Si PIN photodetector substrate.