
- •Contents
- •Preface to the second edition
- •Preface to the first edition
- •1 Special relativity
- •1.2 Definition of an inertial observer in SR
- •1.4 Spacetime diagrams
- •1.6 Invariance of the interval
- •1.8 Particularly important results
- •Time dilation
- •Lorentz contraction
- •Conventions
- •Failure of relativity?
- •1.9 The Lorentz transformation
- •1.11 Paradoxes and physical intuition
- •The problem
- •Brief solution
- •2 Vector analysis in special relativity
- •Transformation of basis vectors
- •Inverse transformations
- •2.3 The four-velocity
- •2.4 The four-momentum
- •Conservation of four-momentum
- •Scalar product of two vectors
- •Four-velocity and acceleration as derivatives
- •Energy and momentum
- •2.7 Photons
- •No four-velocity
- •Four-momentum
- •Zero rest-mass particles
- •3 Tensor analysis in special relativity
- •Components of a tensor
- •General properties
- •Notation for derivatives
- •Components
- •Symmetries
- •Circular reasoning?
- •Mixed components of metric
- •Metric and nonmetric vector algebras
- •3.10 Exercises
- •4 Perfect fluids in special relativity
- •The number density n
- •The flux across a surface
- •Number density as a timelike flux
- •The flux across the surface
- •4.4 Dust again: the stress–energy tensor
- •Energy density
- •4.5 General fluids
- •Definition of macroscopic quantities
- •First law of thermodynamics
- •The general stress–energy tensor
- •The spatial components of T, T ij
- •Conservation of energy–momentum
- •Conservation of particles
- •No heat conduction
- •No viscosity
- •Form of T
- •The conservation laws
- •4.8 Gauss’ law
- •4.10 Exercises
- •5 Preface to curvature
- •The gravitational redshift experiment
- •Nonexistence of a Lorentz frame at rest on Earth
- •The principle of equivalence
- •The redshift experiment again
- •Local inertial frames
- •Tidal forces
- •The role of curvature
- •Metric tensor
- •5.3 Tensor calculus in polar coordinates
- •Derivatives of basis vectors
- •Derivatives of general vectors
- •The covariant derivative
- •Divergence and Laplacian
- •5.4 Christoffel symbols and the metric
- •Calculating the Christoffel symbols from the metric
- •5.5 Noncoordinate bases
- •Polar coordinate basis
- •Polar unit basis
- •General remarks on noncoordinate bases
- •Noncoordinate bases in this book
- •5.8 Exercises
- •6 Curved manifolds
- •Differential structure
- •Proof of the local-flatness theorem
- •Geodesics
- •6.5 The curvature tensor
- •Geodesic deviation
- •The Ricci tensor
- •The Einstein tensor
- •6.7 Curvature in perspective
- •7 Physics in a curved spacetime
- •7.2 Physics in slightly curved spacetimes
- •7.3 Curved intuition
- •7.6 Exercises
- •8 The Einstein field equations
- •Geometrized units
- •8.2 Einstein’s equations
- •8.3 Einstein’s equations for weak gravitational fields
- •Nearly Lorentz coordinate systems
- •Gauge transformations
- •Riemann tensor
- •Weak-field Einstein equations
- •Newtonian limit
- •The far field of stationary relativistic sources
- •Definition of the mass of a relativistic body
- •8.5 Further reading
- •9 Gravitational radiation
- •The effect of waves on free particles
- •Measuring the stretching of space
- •Polarization of gravitational waves
- •An exact plane wave
- •9.2 The detection of gravitational waves
- •General considerations
- •Measuring distances with light
- •Beam detectors
- •Interferometer observations
- •9.3 The generation of gravitational waves
- •Simple estimates
- •Slow motion wave generation
- •Exact solution of the wave equation
- •Preview
- •Energy lost by a radiating system
- •Overview
- •Binary systems
- •Spinning neutron stars
- •9.6 Further reading
- •10 Spherical solutions for stars
- •The metric
- •Physical interpretation of metric terms
- •The Einstein tensor
- •Equation of state
- •Equations of motion
- •Einstein equations
- •Schwarzschild metric
- •Generality of the metric
- •10.5 The interior structure of the star
- •The structure of Newtonian stars
- •Buchdahl’s interior solution
- •10.7 Realistic stars and gravitational collapse
- •Buchdahl’s theorem
- •Quantum mechanical pressure
- •White dwarfs
- •Neutron stars
- •10.9 Exercises
- •11 Schwarzschild geometry and black holes
- •Black holes in Newtonian gravity
- •Conserved quantities
- •Perihelion shift
- •Post-Newtonian gravity
- •Gravitational deflection of light
- •Gravitational lensing
- •Coordinate singularities
- •Inside r = 2M
- •Coordinate systems
- •Kruskal–Szekeres coordinates
- •Formation of black holes in general
- •General properties of black holes
- •Kerr black hole
- •Dragging of inertial frames
- •Ergoregion
- •The Kerr horizon
- •Equatorial photon motion in the Kerr metric
- •The Penrose process
- •Supermassive black holes
- •Dynamical black holes
- •11.6 Further reading
- •12 Cosmology
- •The universe in the large
- •The cosmological arena
- •12.2 Cosmological kinematics: observing the expanding universe
- •Homogeneity and isotropy of the universe
- •Models of the universe: the cosmological principle
- •Cosmological metrics
- •Cosmological redshift as a distance measure
- •The universe is accelerating!
- •12.3 Cosmological dynamics: understanding the expanding universe
- •Critical density and the parameters of our universe
- •12.4 Physical cosmology: the evolution of the universe we observe
- •Dark matter and galaxy formation: the universe after decoupling
- •The early universe: fundamental physics meets cosmology
- •12.5 Further reading
- •Appendix A Summary of linear algebra
- •Vector space
- •References
- •Index

337 |
12.2 Cosmological kinematics: observing the expanding universe |
direction we look. The universe is filled with radiation with a black-body thermal spectrum, with a temperature of 2.725K. The expansion means that the universe has a finite age, or at least that it has expanded in a finite time from a state of very high density. The thermal radiation suggests that the universe was initially much hotter than today, and has cooled as it expanded. The expansion resolves the oldest of all cosmological conundrums, Olbers’ Paradox. The sky is dark at night because we do not receive light from all stars in our infinite homogeneous universe, but only from stars that are close enough for light to have traveled to us during the age of the universe.
But the expansion raises other deep questions, about how the universe evolved to its present state and what it was like much earlier. We would like to know how the first stars formed, why they group into galaxies, why galaxies form clusters: where did the density irregularities come from that have led to the enormously varied structure of the universe on scales smaller than 10 Mpc? We would like to know how the elements formed, what the universe was like when it was too dense and too hot to have normal nuclei, and what the very hot early universe can tell us about the laws of physics at energies higher than we can explore with particle accelerators. We would like to know if the observed homogeneity and isotropy of the universe has a physical explanation.
Answering these questions has led physicists to explore some very deep issues at the frontiers of our understanding of fundamental physics. The homogeneity problem can be solved if the extremely early universe expanded exponentially rapidly, in a phase that physicists call inflation. This could happen if the laws of physics at higher energies than can be explored in the laboratory have a suitable form, and if so this would as a bonus help to explain the density fluctuations that led to the observed galaxies and clusters. As we shall see, it appears that most of the matter in the universe is in an unknown form, which physicists call dark matter because it radiates no light. Even more strangely, the universe seems to be pervaded by a relativistic energy density that carries negative pressure and which is driving the expansion faster and faster; physicists call this the dark energy. The mysteries of dark energy and of inflation may only really be solved with a better understanding of the laws of physics at the highest energies, so theoretical physicists are looking more and more to astronomical answers for clues to better theories.
Modern cosmology is already providing answers to some of these questions, and the answers are becoming more precise and more definite at a rapid pace. This chapter gives a snapshot of the fundamentals of our understanding at the present time (2008). More than any other area covered in this textbook, cosmology is a study that promises new insights, surprises, perhaps even a revolution.
12.2 Co s m o l o g i c a l k i n e m a t i c s : o b s e r v i n g t h e ex p a n d i n g u n i v e r s e
Before we can begin to understand the deep questions of cosmology, let alone their answers, we need to be able to describe and work with the notion of an expanding universe. In this section we develop the metric that describes a homogeneous expanding universe, we

338 |
Cosmology |
show how astronomical observations measure the expansion history, and we develop the framework for discussing physical processes in the expanding universe. In the following section 12.3 we will apply Einstein’s equations to our models to see what GR has to tell us about how the universe expands.
Homogeneity and isotropy of the universe
The simplest approach to applying GR to cosmology is to use the remarkable observed large-scale uniformity. We see, on scales much larger than 10 Mpc, not only a uniform average density but uniformity in other properties: types of galaxies, their clustering densities, their chemical composition and stellar composition. Of course, when we look very far away we are also looking back in time, the time it took the light we observe to reach us; over sufficiently long look-back times we also see evolution, we see a younger universe. But the evolution we see is again the same in all directions, even when we look at parts of the early universe that are very far from one another. We therefore conclude that, on the large scale, the universe is homogeneous. What is more, on scales much larger than 10 Mpc the universe seems to be isotropic about every point: we see no consistently defined special direction.1
A third feature of the observable universe is the uniformity of its expansion: galaxies, on average, seem to be receding from us at a speed which is proportional to their distance from us. This recessional velocity is called the Hubble flow after its discoverer Edwin Hubble. This kind of expansion is easily visualized in the ‘balloon’ model (see Fig. 12.1). Paint regularly spaced dots on a spherical balloon and then inflate it. As it grows, the distance on the surface of the balloon between any two points grows at a rate proportional to that distance. Therefore any point will see all other points receding at a rate proportional to their
Figure 12.1
As the figure is magnified, all relative distances increase at a rate proportional to their magnitudes.
1A universe could be homogeneous but anisotropic, if, for instance, it had a large-scale magnetic field which pointed in one direction everywhere and whose magnitude was the same everywhere. On the other hand, an inhomogeneous universe could not be isotropic about every point, since most – if not all – places in the universe would see a sky that is ‘lumpy’ in one direction and not in another.
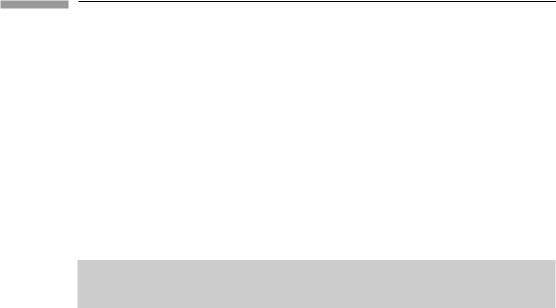
339 |
12.2 Cosmological kinematics: observing the expanding universe |
distance. This proportionality preserves the homogeneity of the distribution of dots with time. It means that our location in the universe is not special, even though we appear to see everything else receding away from us. We are no more at the ‘center’ of the cosmological expansion than any other point is. The Hubble flow is compatible with the Copernican Principle, the idea that the universe does not revolve around (or expand away from) our particular location.
The Hubble expansion gives another opportunity for anisotropy. The universe would be homogeneous and anisotropic if every point saw a recessional velocity larger in, say, the x direction than in the y direction. In our model, this would happen if the balloon were an ellipsoid; to keep its shape it would have to expand faster along its longest axis than along the others. Our universe does not have any measurable velocity anisotropy. Because of this extraordinary simplicity, we can describe the relation between recessional velocity and distance with a single constant of proportionality H:
v = Hd |
(12.1) |
Astronomers call H Hubble’s parameter. Its present value is called Hubble’s constant, H0. The value of H0 is measured – by methods we discuss below – to be H0 = (71 ± 4) km s−1Mpc−1 in astronomers’ peculiar but useful units. To get its value in normal units, convert 1 Mpc to 3.1 × 1022 m to get H0 = (2.3 ± 0.1) × 10−18 s−1. In geometrized units, found by dividing by c, this is H0 = (7.7 ± 0.4) × 10−27 m−1. Associated with the Hubble constant is the Hubble time tH = H0−1 = (4.3 ± 0.2) × 1017 s. This is about 14 billion years, and is the time-scale for the cosmological expansion. The age of the universe will not exactly be this, since in the past the expansion speed varied, but this gives the order of magnitude of the time that has been available for the universe as we see it to have evolved.
We may object that the above discussion ignores the relativity of simultaneity. If the universe is changing in time – expanding – then it may be possible to find some definition of time such that hypersurfaces of constant time are homogeneous and isotropic, but this would not be true for other choices of a time coordinate. Moreover, Eq. (12.1) cannot be exact since, for d > 1.3 × 1026 m = 4200 Mpc, the velocity exceeds the velocity of light! This objection is right on both counts. Our discussion was a local one (applicable for recessional velocities 1) and took the point of view of a particular observer, ourselves. Fortunately, the cosmological expansion is slow, so that over distances of 1000 Mpc, large enough to study the average properties of the homogeneous universe, the velocities are essentially nonrelativistic. Moreover, the average random velocities of galaxies relative to their near neighbors is typically less than 100 km s−1, which is certainly nonrelativistic, and is much smaller than the systematic expansion speed over cosmological distances.
Therefore, the correct relativistic description of the expanding universe is that, in our neighborhood, there exists a preferred choice of time, whose hypersurfaces are homogeneous and isotropic, and with respect to which Eq. (12.1) is valid in the local inertial frame of any observer who is at rest with respect to these hypersurfaces at any location.
The existence of a preferred cosmological reference frame may at first seem startling: did we not introduce special relativity as a way to get away from special reference frames?

340 |
Cosmology |
There is no contradiction: the laws of physics themselves are invariant under a change of observer. But there is only one universe, and its physical make-up defines a convenient reference frame. Just as when studying the solar system it would be silly for us to place the origin of our coordinate system at, say, the position of Jupiter on 1 January 1900, so too would it be silly for us to develop the theory of cosmology in a frame that does not take advantage of the simplicity afforded by the large-scale homogeneity. From now on we will, therefore, work in the cosmological reference frame, with its preferred definition of time.
Models of the universe: the cosmological principle
If we are to make a large-scale model of the universe, we must make some assumption about regions that we have no way of seeing now because they are too distant for our telescope. We should in fact distinguish two different inaccessible regions of the universe.
The first inaccessible region is the region which is so distant that no information (traveling on a null geodesic) could reach us from it no matter how early this information began traveling. This region is everything that is outside our past light-cone. Such a region usually exists if the universe has a finite age, as ours does (see Fig. 12.2). This ‘unknown’ region is unimportant in one respect: what happens there has no effect on the interior of our past light cone, so how we incorporate it into our model universe has no effect on the way the model describes our observable history. On the other hand, our past light cone is a kind of horizon, which is called the particle horizon: as time passes, more and more of the previously unknown region enters the interior of our past light cone and becomes observable. So the unknown regions across the particle horizon can have a real influence on our future. In this sense, cosmology is a retrospective science: it reliably helps us understand only our past.
It must be acknowledged, however, that if information began coming in tomorrow that yesterday’s ‘unknown’ region was in fact very different from the observed universe, say
Figure 12.2
Our location
t = now
Particle horizon
Unknown
Unknown
Unobserved
t = 0
Schematic spacetime diagram showing the past history of the Universe, back to t = 0. The ‘unknown’ regions have not had time to send us information; the ‘unobserved’ regions are obscured by intervening matter.