
- •VOLUME 2
- •CONTRIBUTOR LIST
- •PREFACE
- •LIST OF ARTICLES
- •ABBREVIATIONS AND ACRONYMS
- •CONVERSION FACTORS AND UNIT SYMBOLS
- •CARBON.
- •CARDIAC CATHETERIZATION.
- •CARDIAC LIFE SUPPORT.
- •CARDIAC OUTPUT, FICK TECHNIQUE FOR
- •CARDIAC OUTPUT, INDICATOR DILUTION MEASUREMENT OF
- •CARDIAC PACEMAKER.
- •CARDIAC OUTPUT, THERMODILUTION MEASUREMENT OF
- •CARDIOPULMONARY BYPASS.
- •CARDIOPULMONARY RESUSCITATION
- •CARTILAGE AND MENISCUS, PROPERTIES OF
- •CATARACT EXTRACTION.
- •CELL COUNTER, BLOOD
- •CELLULAR IMAGING
- •CEREBROSPINAL FLUID.
- •CHEMICAL ANALYZERS.
- •CHEMICAL SHIFT IMAGING.
- •CHROMATOGRAPHY
- •CO2 ELECTRODES
- •COBALT-60 UNITS FOR RADIOTHERAPY
- •COCHLEAR PROSTHESES
- •CODES AND REGULATIONS: MEDICAL DEVICES
- •CODES AND REGULATIONS: RADIATION
- •COGNITIVE REHABILITATION.
- •COLORIMETRY
- •COMPUTERS IN CARDIOGRAPHY.
- •COLPOSCOPY
- •COMMUNICATION AIDS FOR THE BLIND.
- •COMMUNICATION DEVICES
- •COMMUNICATION DISORDERS, COMPUTER APPLICATIONS FOR
- •COMPOSITES, RESIN-BASED.
- •COMPUTED RADIOGRAPHY.
- •COMPUTED TOMOGRAPHY
- •COMPUTED TOMOGRAPHY SCREENING
- •COMPUTED TOMOGRAPHY SIMULATOR
- •COMPUTED TOMOGRAPHY, SINGLE PHOTON EMISSION
- •COMPUTER-ASSISTED DETECTION AND DIAGNOSIS
- •COMPUTERS IN CARDIOGRAPHY.
- •COMPUTERS IN THE BIOMEDICAL LABORATORY
- •COMPUTERS IN MEDICAL EDUCATION.
- •COMPUTERS IN MEDICAL RECORDS.
- •COMPUTERS IN NUCLEAR MEDICINE.
- •CONFOCAL MICROSCOPY.
- •CONFORMAL RADIOTHERAPY.
- •CONTACT LENSES
- •CONTINUOUS POSITIVE AIRWAY PRESSURE
- •CONTRACEPTIVE DEVICES
- •CORONARY ANGIOPLASTY AND GUIDEWIRE DIAGNOSTICS
- •CRYOSURGERY
- •CRYOTHERAPY.
- •CT SCAN.
- •CUTANEOUS BLOOD FLOW, DOPPLER MEASUREMENT OF
- •CYSTIC FIBROSIS SWEAT TEST
- •CYTOLOGY, AUTOMATED
- •DECAY, RADIOACTIVE.
- •DECOMPRESSION SICKNESS, TREATMENT.
- •DEFIBRILLATORS
- •DENTISTRY, BIOMATERIALS FOR.
- •DIATHERMY, SURGICAL.
- •DIFFERENTIAL COUNTS, AUTOMATED
- •DIFFERENTIAL TRANSFORMERS.
- •DIGITAL ANGIOGRAPHY
- •DIVING PHYSIOLOGY.
- •DNA SEQUENCING
- •DOPPLER ECHOCARDIOGRAPHY.
- •DOPPLER ULTRASOUND.
- •DOPPLER VELOCIMETRY.
- •DOSIMETRY, RADIOPHARMACEUTICAL.
- •DRUG DELIVERY SYSTEMS
- •DRUG INFUSION SYSTEMS
21.Kass L. Staining of Granulocytic Cells by Chlorazol Black E. Am J Clin Pathol 1981;76:810–812.
22.ADVIA 2120 Operator’s Guide V1.0.1.00, 2004. Bayer HealthCare LLC, Diagnostics Division, Tarrytown, NY.
23.Harris N, Kunicka J, Kratz A. The ADVIA 2120 Hematology System: Flow-cytometry-based analysis of blood and body fluids in the routine hematology laboratory. Lab Hematol 2005;11(1):47–61.
24.Fernandez T, et al. Performance Evaluation of the Coulter LH 750 Hematology Analyzer. Lab Hematol 2001;7:217–228.
25.Aulesa C, et al. Validation of the Coulter LH 750 in a Hospital Reference Laboratory. Lab Hematol 2003;9:15–28.
26.Coulter VCS Technology: Clinical Case Studies. Beckman Coulter Bulletin No. 3008.
27.Coulter Gen S System Enhanced VCS Technology: Clinical Case Studies. Bulletin 9165.
28.Fujimoto K. Principles of Measurement in Hematology Analyzers Manufactured by Sysmex Corporation. Sysmex J Inter 1999;9(1):31–44.
29.Sakata et al. (to Toa Medical Electronics Co, Ltd.); Reagent And Method For Classifying Leukocytes By Flow Cytometry. US Patent 5,928,949. 1999 July 27.
30.Uchihashi et al. (Sysmex Corporation); Reagent For Measurement of Leukocytes And Hemoglobin Concentration In Blood. US Patent 5,968,832. 1999 Oct 19.
Further Reading
Shibata et al. (to Toa Medical Electronics Co, Ltd.); Method And Apparatus For Determining A Particle Criterion And Particle Analyzer Using The Criterion. US Patent 5,690,105. 1997 Nov 25.
Sakata et al. (to Sysmex Corporation); Method For Classifying And Counting Immature Leukocytes. US Patent 5,958,776. 1999 Sept 28.
Shibata et al. (to Sysmex Corporation); Reagent and Method For Classification And Counting Of Leukocytes. US Patent 6,004,816. 1999 Dec 21.
Ruzicka K, et al. The New Hematology Analyzer Sysmex XE-2100: Performance Evaluation of a Novel White Blood Cell Differential Technology. Arch Pathol Lab Med 2001;125: 391–396.
Walters J, Garrity P. Performance Evaluation of the Sysmex XE2100 Hematology Analyzer. Lab Hematol 2000;6:83–92.
Briggs C, et al. Performance Evaluation of the Sysmex XE-2100 Automated Haematology Analyzer. Sysmex J Inter 1999;9(2): 113–119.
See also BLOOD COLLECTION AND PROCESSING; CELL COUNTERS, BLOOD;
CYTOLOGY, AUTOMATED.
DIFFERENTIAL TRANSFORMERS. See LINEAR
VARIABLE DIFFERENTIAL TRANSFORMERS.
DIGITAL ANGIOGRAPHY
JAMES R. BENNETT
University of Iowa
Iowa City, Iowa
INTRODUCTION
The term ‘‘angiography’’ is derived from the Greek angeio-, meaning blood vessel, and graphein, meaning representation of a specified object (1). Thus, a very general definition
DIGITAL ANGIOGRAPHY |
421 |
of angiography would be ‘‘the representation of blood vessels’’. Angiography is a technique that allows visualization of any aspect of the human circulatory system. The principle of angiography is to increase the conspicuity of blood vessels during imaging by displacing the blood within the vessels of interest with a contrast medium, although this definition does not necessarily hold true for all imaging modalities. Whether it is the venous system, cardiac arteries, or the abdominal aorta, whenever diagnostic information is needed on a patient’s vasculature, some form of angiography is likely to be employed. This article will be structured as follows: a brief history of angiography, the advent of digital subtraction angiography (DSA), noncatheter/noninvasive angiographic techniques, and finally a discussion on the future of vascular imaging.
A BRIEF HISTORY AND OVERVIEW
The first angiography was performed shortly after Wilhelm Roentgen’s discovery of the X ray. In January of 1896, Mr. Hascheck and Dr. Lindenthal, of the Physicochemical Institute in Vienna, produced the first angiogram by injecting Teichmann’s mixture into the arteries of a cadaver’s hand and imaging the hand using X rays, shown in Fig. 1 (2). Born from this experiment was the field of vascular imaging, and for the next 90 years, angiography was the field’s principal technique. In this section, the explanation of angiography are described in terms of X-ray imaging for the sake of simplicity. The overall principles of X-ray angiography generally hold true for all modalities. Yet, there are important deviations when utilizing other imaging technology, which are noted and explained in subsequent sections.
The principle behind all radiographic imaging is differential X-ray attenuation. As an X-ray beam passes through an object, the intensity of the beam is attenuated, or diminished, in proportion to the density and thickness of the object. This attenuation can be modeled by the following equation:
I ¼ I0e mt
where I is the X-ray intensity after the original X-ray beam, with intensity I0, passes through an object with a thickness of t and a linear attenuation coefficient of m. For this article, assume that beam attenuation is logarithmically proportional to the attenuation coefficient, which in turn is linearly proportional to the material density.
Returning to radiographic imaging, materials with different density attenuate X-rays to differing degrees. Thus, a radiographic image is formed when an X-ray beam passes through an object, and is then captured on the other side by a radiosensitive fluorescent screen. The screen changes based on the X-ray intensity: The more intense the X-ray beam is, the greater the screen fluoresces and visa versa. However, there must be significant differences in material density to produce an image. For example, the femur (bone) is easily identified in an X-ray study of the leg, but differentiation between skin and muscle is nearly impossible. This is due to the fact that bone is significantly denser than the surrounding tissue: Skin and muscle have similar densities, and therefore attenuate X rays in a
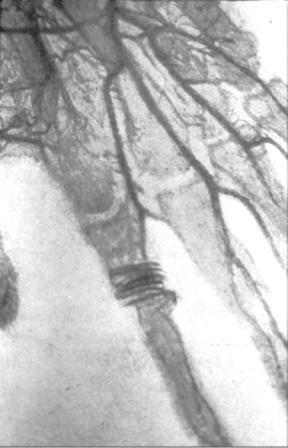
422 DIGITAL ANGIOGRAPHY
Figure 1. Radiograph of cadaver hand with contrast injection. (Copyrighted # by Radiology Centennial, Inc.)
nearly identical fashion. This attenuation homogeneity is true for many tissues in the body, including blood and blood vessels; hence the need for a dense contrast medium to discriminate the blood vessels from the adjacent tissues.
In Fig. 1, Teichmann’s mixture was used as a contrast medium to image the blood vessels of a cadaver’s hand. Teichmann’s mixture is a dense liquid containing lime, mercury, and petroleum (2). Injection of the mixture displaced the blood within the vessels; as the X-ray beam passes through the hand, the material within the vessels significantly attenuates the beam, and results in the visualization of the vessels in the resultant image. For the next 90 years, the main changes to this technique would be the contrast material and its delivery technique. Iodinated agents have become the main contrast medium for X-ray angiography. Furthermore, catheter guided injections became the predominate means to deliver a contrast injection in a localized region of interest (ROI). This technique utilizes a small puncture to a main artery, where a guide wire is inserted and routed through the arterial system and parked at a location proximal (upstream) of the ROI, where the contrast agent is delivered. This technique will be explored further in the subsequent section.
The field of radiology has arguably incurred the greatest paradigm shift of any medical field resulting from the
introduction of practical computing technology. Research in digital imaging began in the late 1960s and early 1970s and clinical applications were realized in the 1980s. Twodimensional (2D) X-ray imaging was the first digital application on the clinical radiology scene. In a general sense, film was replaced with the digital capture of X-ray images. This change to digital imaging brought vast and immediately benefits, essentially falling into three categories: time, money, and quality. Digital imaging created near instantaneous X-ray images, eliminating the costly and time consuming process of developing film. Relative to angiography, this allowed radiologists to immediately ascertain whether or not the contrast injection was adequately captured during imaging, which was previously delayed by the lengthy film developing process. Postprocessing of the digital images had a revolutionary effect on image quality. Although the resolution of the digital images was about a fivefold decrease relative to film, the ability to digitally manipulate images far outweighed this loss in resolution. Radiologists could instantly adjust the contrast and brightness of an image, yet the most significant gain in the quality of angiographic imaging was the advent of digital subtraction angiography.
DIGITAL SUBTRACTION ANGIOGRAPHY
Digital subtraction angiography (DSA) undoubtedly revolutionized angiography and the field of vascular imaging as a whole. This is currently the gold standard against which all emerging vascular imaging techniques are compared, however, subtraction imaging is not a new technique to the field of radiology. It was first theorized in the 1930s that if a radiograph were taken before a contrast injection and another as the contrast passes through the imaging window, it would be possible to subtract out everything but the contrast within the vessels (3). The goal of subtraction is to remove the nondiagnostic artifacts in the X-ray image; organs, bones, surgical staples, metallic implants, and other fairly dense objects that can overshadow the contrast enhanced vessels as they intersect within the imaging plane. Essentially, when the enhanced vessel crosses a dense object, it becomes indistinguishable from the artifact on the radiographic image and can significantly impede interpretation. Manual film-subtraction angiography proved to be extremely valuable to the field of vascular imaging. Yet this process is expensive and time consuming: a single subtracted image involves complicated film development that usually requires multiple attempts before a usable image is produced.
The key principle behind image subtraction is the acquisition of a preinjection image, or mask image. Essentially, if two images are taken of the same motionless object, and the first image is subtracted from the second, the resultant image should be blank. If, however, there is any change to the object between the two image captures, only the difference will be visible when the first image is subtracted from the second. For example, in Fig. 2a, there is an image of an apple. Figure 2b represents the same apple, taken with the same camera in the same position, but with an
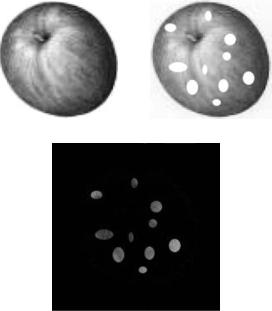
interval of several days in between the two images. Within this time interval, the apple became spotted, while its shape did not change. Each image is a grayscale matrix of 200 200 pixels; each pixel has a grayscale value from 0 to 255. The first image matrix, or mask matrix, will be denoted by M and the second image will be denoted by I. To subtract the two images, it is necessary to subtract each matrix entry in the second image from its corresponding entry in the mask image, giving the subtracted image matrix, S, represented by this formula:
S ¼ I M
The resultant image, S, displays only the spots on the apple, while everything else in the image is black. Black represents zero on the grayscale image, thus all points in the two image matrices that did not become zero when the images are subtracted. The next step is to equalize the histogram of the resultant image.
A histogram is a plot representing the relative occurrence of pixel values in an image; the x axis contains the pixel values, 0–255, and the y axis contains the number of times that pixel value occurs in the image. An image that is very dark will have a histogram that is weighted toward the origin, as black would be the predominant pixel value and is represented by zero on grayscale images. Very light images are weighted toward the outer boundary because white is represented by 255. Upon close examination, the subtracted image in Fig. 2c does not exactly match the change in the two previous images. White spots were added to the second image and the subtracted image shows similar spots, but not perfectly white spots. It is necessary to adjust the histogram of the image after subtraction of the two images, due to the fact that subtraction does not result
Figure 2. (a) Original picture of apple. (b). Apple after time elapsed and became spotted. (c) Digital subtraction of Fig. 2a from 2b.
DIGITAL ANGIOGRAPHY |
423 |
in an image with an equalized histogram. An image with an equalized histogram has pixel values that are spread throughout the histogram. Figure 3a represents the histogram of the original apple image (Fig. 2a). The background of the image is white, and therefore the histogram is weighted toward the outer boundary. However, there is also a fairly equal spread of pixel values throughout the entire range. In comparison, Fig. 3b shows the histogram of the subtracted apple image (Fig. 2c). This histogram weighted heavily toward the origin and does not have pixel values throughout the entire range. The reason for this compressed histogram is beyond the scope of this article, but it is a general rule that the resultant image from subtraction will have a compressed histogram. The next step is to utilize an algorithm that equalizes the histogram by spreading the pixel values out over the entire range. Figure 3d shows the subtracted image after its histogram (Fig. 3c) was equalized. Now, the subtracted image is a true representation of the change in the original image.
Digital subtraction works well in optimal conditions where the camera and object do not move within the interval that the two images are taken. However, realworld implementation presents a host of issues, the most prevalent being patient motion. Imaging equipment is generally very precise in its positioning, that is, it does not deviate from its expected location. Patients tend to shift position in between the contrast and mask image capture, the side effect of which is the introduction of artificial artifacts in the subtracted image. An example of such artifact introduction is given in Fig. 4, where one of the two images has been shifted to the upper right quadrant. In some cases, the patient motion is due to discomfort, as the contrast medium tends to displace oxygenated blood within the vessels that may result in a burning sensation. Another source of patient motion can be organ movement, which can similarly introduce motion. In either case, motion results in image artifacts that can impede diagnostic interpretation. It is possible to compensate for these movements, given that the movement is a linear translation within the imaging plane. Simply shifting the contrast and mask images can realign the objects within the image, which will minimize the induced artifacts. In film-based image subtraction, this shifting can require multiple attempts before a usable image is produced because there is much time involved with developing the subtracted image. However, with digital imaging, it is possible to instantly manipulate the position of the two images. This technique is termed pixel shifting, as one generally shifts the images, pixel by pixel, until the objects are aligned within the images. Unfortunately, a significant amount of patient movement does not occur linearly within the imaging plane. Movement is nonlinear if the patient rolls their body, extends their limb, or any movement that is not translational within the imaging plane (the patient table can be representative of the imaging plane in most cases). A technique has recently been developed to adjust for such nontranslation movement. Although the details are beyond the scope of this article, essentially a map is created of objects both within the mask and contrast images. An algorithm compares the two maps and adjusts, or stretches, the images based on differences within the maps.
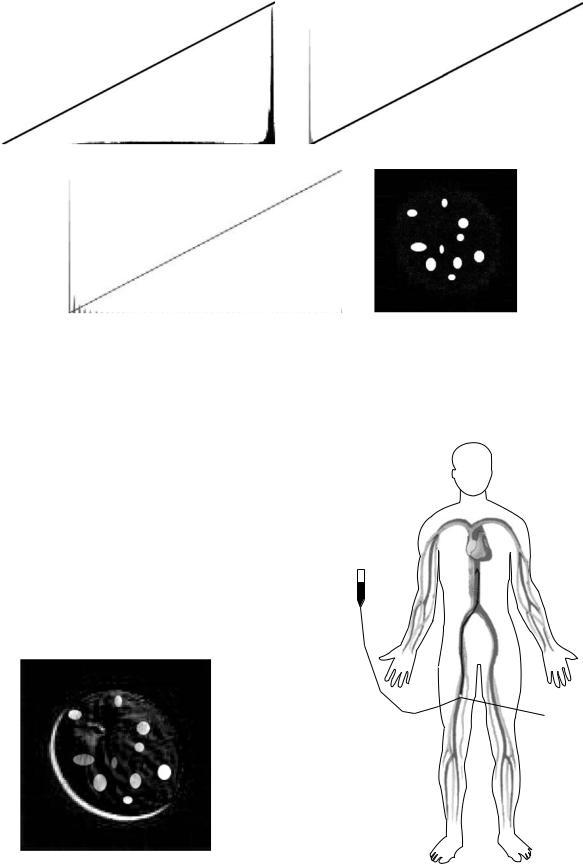
424 DIGITAL ANGIOGRAPHY
Figure 3. Histogram of Fig. 2a. (b) Histogram of Fig. 2c. (c) Histogram of Fig. 3d. (d) Figure 2c after histogram equalization algorithm applied.
Now that digital subtraction imaging has been covered, a brief explanation of DSA follows. A typical DSA procedure begins with the insertion of a catheter in the femoral artery, as shown in Fig. 5. A guidewire is inserted through the catheter and routed to a position proximal (upstream) to the vessels of interest, through which a contrast delivery sheath is inserted. The next step depends on the purpose of the study: if the clinician solely wishes to view one particular area, for example, the abdominal aorta, they will take a single mask image, inject and image the contrast, and then perform digital subtraction. If, however, the clinician wishes to perform a runoff sequence, where the peripheral vasculature is studied, they will program the imaging equipment to follow a series of imaging stations. These stations are required because the desired image is significantly larger than the actual imaging window. Thus, many frames, or stations, overlap each other and follow the vessels of interest until their termination at the extremities. Once
the stations are programmed, the imaging apparatus steps through the programmed sequence and captures a mask image at each station. Next, the machine returns to the first station,and whentheclinician isready toinject thecontrast,
Contrast
injector
Catheter access puncture
Figure 4. Example of digital subtraction when object shifts |
|
between original and mask frame. |
Figure 5. Diagram of DSA contrast delivery technique. |
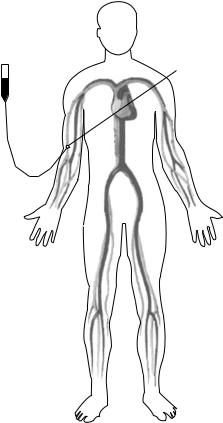
the machine begins imaging the first station at a set capture rate (typically 3–5 frames s). The clinician injects the contrast and watches the viewing monitor for the contrast arrival at the first station. When the contrast reaches the lowerportionofthefirststation,theytriggers themachineto advance to the next station, which is always distal (downstream) to the previous station. Once the contrast passes through the second station, the clinician triggers the machine to the next station, and this process is repeated until the contrast reaches the final station. Digital subtraction is performed at each station, and the final step is merging the subtracted images from each station into a single image of the patient’s peripheral vasculature. One of the most recent advances in DSA has come through flat panel technology. In traditional X-ray imaging systems, the X-ray beam is passed through the patient and captured with a scintillator, which is a screen that fluoresces in proportion to X-ray intensity. Typically, a camera captures the luminescence from the screen and transfers this image digitally to a computer. Flat panel technology replaces this system with complementary metal oxide semiconductor (CMOS) detector panels, which can be thought of as radiosensitive charge coupled device (CCD) chips. Essentially, the panel is a matrix of radiosensitive pixels, which monitor the X-ray beam intensity at each pixel. The intensity level from each pixel is converted to a digital signal, which is then reconstructed to form an X-ray image. This system bypasses the need for the scintillator screen and camera setup by capturing the X-ray beam directly. The benefits of flat panel technology are an increase in resolution and elimination of geometric distortion resulting from the nature of the optical camera system. Another advance in DSA technology is three-dimensional (3D) imaging, where the imaging system rotates around the patient table, capturing images at different angles, from which 3D images are reconstructed.
NON-CATHETER/NONINVASIVE ANGIOGRAPHY
Non-catheter/noninvasive imaging technologies, especially 3D technologies, have created the next paradigm shift in vascular imaging. The principles of such imaging technologies will not be discussed in this article, as such information can be found in this Encyclopedia within the respective modality articles. This section will begin with single detec- tor-row computed tomography (CT) X-ray imaging, which became commercially available in the 1980s. This technology was able to reconstruct 2D axial slices (images) of the patient. In a similar technique to DSA, iodinated contrast agents were injected into the vasculature during CT imaging, which produced basic 2D contrast enhanced vascular images. This technique could be considered fairly rudimentary as compared to today’s standards. Yet, it did allow clinicians to view patient’s vasculature in relation to the rest of the body, instead of the enhanced vasculature being superimposed upon the rest of the body, as in DSA. As CT advanced, so did CT technology. Soon, multirow detector arrays were introduced to CT, which revolutionized vascular imaging. Multirow detectors increased longitudinal coverage and image resolution. It allowed for 3D images to
DIGITAL ANGIOGRAPHY |
425 |
Contrast
injector Intravenous(IV) access
Figure 6. Diagram of computed tomography angiography (CTA) and gadolinium enhanced magnetic resonance angiography (MRA) contrast delivery technique.
be produced, along with increased visualization of small vessels. However, the greatest contribution of multirow detectors was the increased scanning speed. As scan time decreased, conspicuity increased because patient motion, and therefore the resulting artifacts, was less prevalent. Further benefits resulted from the noninvasive nature of this technique. Instead of catheter based contrast delivery in DSA, the iodinated contrast is injected intravenously, as shown in Fig. 6. The contrast travels from the venous system to the right side of the heart, through the pulmonary system, and then back to the left side of the heart where it is pumped throughout the arterial system. The contrast can be imaged wherever diagnostic information of vessels is needed. This technology greatly decreased the time and cost associated with an angiographic study, along with providing superior vascular images. There are several drawbacks associated with CT imaging. It requires an increased amount of radiation and iodinated contrast dose when compared with 2D angiography. The U.S. Food and Drug Administration (FDA) has recently classified ionizing radiation, used to create X-ray images, as a carcinogen. Computed tomography imaging delivers a substantial dose of ionizing radiation, especially in a full-body scan. The iodinated contrast material is nephrotoxic. In cases where a patient has borderline kidney function, the toxicity of a large dose of iodinated contrast may induce renal failure.
426 DIGITAL ANGIOGRAPHY
Another drawback to CT imaging is that metallic implants, such as hip prostheses, create artifacts in the images. Finally, calcium within the vasculature can limit evaluation of vessel patency (openness).
Magnetic resonance (MR) imaging can also be used to perform diagnostic imaging of blood vessels. To fully comprehend the nature of this modality, and therefore MR angiography, it would be recommended to review the article on MR imaging in this Encyclopedia. There are many methods for imaging the vasculature with this modality: time of flight (tof) angiography, phase-contrast (PC) angiography, and gadolinium enhanced angiography. In this section, tof and gadolinium enhanced MR angiography will be discussed, as they are the most prevalent in clinical use. Time of flight angiography utilizes constant radio frequency (RF) pulses within a presaturation slab. The RF pulses do not allow the atomic spins within that slab to realign with the magnetic field. Thus, these saturated atoms give off a very weak signal. However, when fresh blood from the arteries enters the imaging slice, the RF pulses have not saturated the spins, and therefore it gives off a much stronger signal when passing through the imaging slice. This technique works well for arterial visualization, the only drawback is that this technique does not work when the vessel, and therefore blood flow, is parallel with the imaging slice or if the blood flow is slow or turbulent. This is due to the fact that the blood becomes saturated as it travels within the saturation slab, and therefore does not give a strong signal, unlike the fresh blood (4). Gadolinium enhanced MRA does not have any of these drawbacks associated with tof angiography. Gadolinium enhanced MRA works in a similar fashion as DSA or CTA; however, the physics behind its principles are quite dissimilar. Gadolinium contrast agents work by shortening the T1 time of the blood and blood vessels (4). This enhances the blood and blood vessel visualization as the contrast enhanced blood passes through the imaging slice. Recently, there has been an emergence of advanced MRA techniques, such as fresh blood imaging and timeresolved MRA, which are well beyond the scope of this article. Magnetic resonance angiography overcomes many of the drawbacks associated with CTA; however, it is not without its own issues. Patients with pacemakers, surgical clips, metallic prostheses, or foreign bodies cannot undergo an MRA examination. Also, patients with claustrophobia cannot undergo MR imaging. It has a longer scan time as compared to CT imaging, and finally it does not produce images with adequate vessel wall definition.
Ultrasound (US) plays an important role in vascular imaging. Although most applications of this technique would not generally be classified as angiography, it is an extremely important tool nonetheless. The latest widescale medical application of ultrasound is termed triplex imaging. Triplex imaging combines grayscale, color, and Doppler information to form images of the vasculature with color coded Doppler blood flow data overlaid on the grayscale image. One of many recent developments in US imaging is intravascular ultrasound (IVUS). This technique works by inserting an extremely small US transducer into the blood vessel of interest via a catheter. The IVUS produces the most accurate vessel wall characterization of any
currently available modality. Another recently developed technique involves injecting microbubbles into the bloodstream. This US technique would be the most likely to be classified as angiography. The principle behind microbubble US is that these tiny bubbles will create differences in density in the blood, which can be easily detected by a US transducer. Both IVUS and the microbubble technique are currently in their infantile stages, but have vast potential to improve vascular imaging. There are many advantages utilizing US as a vascular imaging modality, including the low cost and portability relative to MR and CT scanners. Also, vascular US imaging does not involve any harmful contrast medium or ionizing radiation.
FUTURE OF VASCULAR IMAGING
The need for vascular imaging will not diminish in the foreseeable future. The United States alone has seen obesity and diabetes climb to a near epidemic scale, factors that can significantly increase the incidence of circulatory disease. According to the World Health Organization, in 1997 there were 15.3 million deaths due to circulatory disease worldwide. It is unknown whether these deaths could have been prevented with medical intervention, but the main point is that circulatory disease will not be subsiding in the near future. Thus, it is probable that there will be an increasing need for diagnostic vascular imaging. There are many promising emergent modalities and techniques that will be included in the next generation of vascular imaging. Improvements such as 3D dimensional ultrasound and 256-row CT scanners are not far from implementation. One improvement that could have a vast impact on vascular imaging would be a nonnephrotoxic radioopaque contrast agent. The optimal solution would be a modality that combines the resolution and detail provided with CTA with the relatively risk-free imaging found in ultrasound and MRA.
BIBLIOGRAPHY
1.Merriam-Webster Online Dictionary. (2005). Merriam Webster Online. [Online]. Merriam-Webster. Available at http:// www. merriam-webster.com Accessed 2005 April 18.
2.Sprawls P. 1996 Feb 1. The X-ray Century. [Online]. Emory University. Available at http://www.emory.edu/X-RAYS/century. htm. Accessed 2005; May 3.
3.Ziedses des Plantes B. Plantinigraphie en subtractie Roentgenographische differentiatiemethoden. Ph. D. dissertation, University of Utrecht; 1934.
4.Bakal CW, Silberzweig JE, Cynamon J, Sprayregen S. Vascular and Interventional Radiology: Principles and Practice. New York: Thieme Medical Publishers; 2002.
Reading List
Hagspiel KD, Matsumoto AH. The Radiological Clinics of North America. Philadelphia: W. B. Saunders, 2002.
Gonzalez RC, Woods RE. Digital Image Processing. Upper Saddle River (NJ): Prentice Hall; 2002.
World Health Organization. The World Health Report 1998. Geneva, Switzerland: World Health Organization; 1998.