
- •VOLUME 2
- •CONTRIBUTOR LIST
- •PREFACE
- •LIST OF ARTICLES
- •ABBREVIATIONS AND ACRONYMS
- •CONVERSION FACTORS AND UNIT SYMBOLS
- •CARBON.
- •CARDIAC CATHETERIZATION.
- •CARDIAC LIFE SUPPORT.
- •CARDIAC OUTPUT, FICK TECHNIQUE FOR
- •CARDIAC OUTPUT, INDICATOR DILUTION MEASUREMENT OF
- •CARDIAC PACEMAKER.
- •CARDIAC OUTPUT, THERMODILUTION MEASUREMENT OF
- •CARDIOPULMONARY BYPASS.
- •CARDIOPULMONARY RESUSCITATION
- •CARTILAGE AND MENISCUS, PROPERTIES OF
- •CATARACT EXTRACTION.
- •CELL COUNTER, BLOOD
- •CELLULAR IMAGING
- •CEREBROSPINAL FLUID.
- •CHEMICAL ANALYZERS.
- •CHEMICAL SHIFT IMAGING.
- •CHROMATOGRAPHY
- •CO2 ELECTRODES
- •COBALT-60 UNITS FOR RADIOTHERAPY
- •COCHLEAR PROSTHESES
- •CODES AND REGULATIONS: MEDICAL DEVICES
- •CODES AND REGULATIONS: RADIATION
- •COGNITIVE REHABILITATION.
- •COLORIMETRY
- •COMPUTERS IN CARDIOGRAPHY.
- •COLPOSCOPY
- •COMMUNICATION AIDS FOR THE BLIND.
- •COMMUNICATION DEVICES
- •COMMUNICATION DISORDERS, COMPUTER APPLICATIONS FOR
- •COMPOSITES, RESIN-BASED.
- •COMPUTED RADIOGRAPHY.
- •COMPUTED TOMOGRAPHY
- •COMPUTED TOMOGRAPHY SCREENING
- •COMPUTED TOMOGRAPHY SIMULATOR
- •COMPUTED TOMOGRAPHY, SINGLE PHOTON EMISSION
- •COMPUTER-ASSISTED DETECTION AND DIAGNOSIS
- •COMPUTERS IN CARDIOGRAPHY.
- •COMPUTERS IN THE BIOMEDICAL LABORATORY
- •COMPUTERS IN MEDICAL EDUCATION.
- •COMPUTERS IN MEDICAL RECORDS.
- •COMPUTERS IN NUCLEAR MEDICINE.
- •CONFOCAL MICROSCOPY.
- •CONFORMAL RADIOTHERAPY.
- •CONTACT LENSES
- •CONTINUOUS POSITIVE AIRWAY PRESSURE
- •CONTRACEPTIVE DEVICES
- •CORONARY ANGIOPLASTY AND GUIDEWIRE DIAGNOSTICS
- •CRYOSURGERY
- •CRYOTHERAPY.
- •CT SCAN.
- •CUTANEOUS BLOOD FLOW, DOPPLER MEASUREMENT OF
- •CYSTIC FIBROSIS SWEAT TEST
- •CYTOLOGY, AUTOMATED
- •DECAY, RADIOACTIVE.
- •DECOMPRESSION SICKNESS, TREATMENT.
- •DEFIBRILLATORS
- •DENTISTRY, BIOMATERIALS FOR.
- •DIATHERMY, SURGICAL.
- •DIFFERENTIAL COUNTS, AUTOMATED
- •DIFFERENTIAL TRANSFORMERS.
- •DIGITAL ANGIOGRAPHY
- •DIVING PHYSIOLOGY.
- •DNA SEQUENCING
- •DOPPLER ECHOCARDIOGRAPHY.
- •DOPPLER ULTRASOUND.
- •DOPPLER VELOCIMETRY.
- •DOSIMETRY, RADIOPHARMACEUTICAL.
- •DRUG DELIVERY SYSTEMS
- •DRUG INFUSION SYSTEMS
67.Redford G, Clegg RB. Real-Time Fluorescence Lifetime Imaging and FRET using Fast Gated Image Intensifiers. In: Periasamy A, Day RN, editors. Molecular Imaging: FRET Microscopy and Spectroscopy. New York: Oxford University Press; 2005. Chapt. 11, in press.
68.Bastiaens PI, Squire A. Fluorescence lifetime imaging microscopy: spatial resolution of biochemical processes in the cell. Trends Cell Biol 1999;9:48–52.
69.Hohng S, Joo C, Ha T. Single-Molecule Three-Color FRET. Biophys J 2004;87(2):1328–1337.
70.Petersen NO, Hoddelius PL, Wiseman PW, Seger O, Magnusson KE. Quantitation of membrane receptor distributions by image correlation spectroscopy: concept and application. Biophys J 1993;65(3):1135–1146.
71.Cheng JX, Volkmer A, Book LD, Xie XS. Multiplex coherent anti-Stokes Raman scattering microspectroscopy and study of lipid vesicles. J Phys Chem B 2002a 106:8493–8498.
72.Cheng JX, Jia YK, Zheng G, Xie XS. Laser-scanning coherent anti-Stokes Raman scattering microscopy and applications to cell biology. Biophys J 2002b;83(1):502–509.
73.Carey PR. Raman spectroscopy, the sleeping giant in structural biology, awakes. J Biol Chem 1999;274(38):26625–26628.
74.Salmaso BL, Puppels GJ, Caspers PJ, Floris R, Wever R, Greve J. Resonance Raman microspectroscopic characterization of eosinophil peroxidase in human eosinophilic granulocytes. Biophys J 1994;67(1):436–446.
75.Puppels GJ, de Mul FF, Otto C, Greve J, Robert-Nicoud M, Arndt-Jovin DJ, Jovin TM. Studying single living cells and chromosomes by confocal Raman microspectroscopy. Nature (London) 1990;347(6290):301–303.
76.Volkmer A, Cheng JX, Xie XS. Vibrational imaging with a high sensitivity via epidetected coherent anti-Stokes Raman scattering microscopy. Phys Rev Lett 2001;87:023901.
77.Uzunbajakava N, Lenferink A, Kraan Y, Volokhina E, Vrensen G, Greve J, Otto C. Nonresonant confocal Raman imaging of DNA and protein distribution in apoptotic cells. Biophys J 2003;84(6):3968–3981.
78.Hawi SR, Rochanakij S, Adar F, Campbell WB, Nithipatikom K. Detection of membrane-bound enzymes in cells using immunoassay and Raman microspectroscopy. Anal Biochem 1998;259(2):212–217.
79.Diem M, Chiriboga L, Lasch P, Pacifico A. IR spectra and IR spectral maps of individual normal and cancerous cells. Biopolymers 2002;67(4–5):349–353.
80.Nan X, Yang WY, Xie XS. CARS microscopy: lights up lipids in living cells. Biophoton Int 2004;11(8):44–47.
See also CYTOLOGY, AUTOMATED; MICROSCOPY, CONFOCAL; MICROSCOPY,
ELECTRON.
CEREBROSPINAL FLUID. See HYDROCEPHALUS, TOOLS
FOR DIAGNOSIS AND TREATMENT OF.
CHEMICAL ANALYZERS. See ANALYTICAL METHODS,
AUTOMATED.
CHEMICAL SHIFT IMAGING. See NUCLEAR
MAGNETIC RESONANCE SPECTROSCOPY.
CHROMATOGRAPHY
THAYNE L. EDWARDS
University of Washington
Seattle, Washington
CHROMATOGRAPHY 101
INTRODUCTION
Chromatography is the process of separating a mobile phase mixture into its individual components using the relative interactions of the components with a stationary phase. Chromatography is often used as a method of purification, even when the components are closely related. When used in conjunction with a concentration or massbased sensor, it can also be a power analytical method. This chapter explores the basic processes of the various types of chromatography and the closely related techniques of fieldflow fractionation (FFF) and electrophoresis. Some of the basic theory of chromatography will be given in terms of retention mechanism and separation performance. In addition, a description of the basic types of chromatography, electrophoresis, and FFF will be given in relation to their theory and application.
Chromatography literally means ‘‘color writing’’ because of an observation in the early 1900s by Mikhail Tswett in separating pigments of plants into various color bands using CaCO3 (1). Although Tswett is considered the father of chromatography, he was not the first to observe the chromatographic process in an experimental setting. Pliny the Elder (ca. 79 AD) recorded a crude paper chromatography experiment. Several others between this time and the realization of the usefulness of chromatography by Tswett also observed the process in the laboratory. It was not until the 1930s that it was recognized generally as an analytical technique (2). Since then, many advances, discoveries, and inventions have made chromatography an indispensable laboratory and industrial technique.
The purpose of chromatographic methods falls under either purification or analysis. Gas chromatography is almost invariably an analytical method whereas liquid chromatography is used for either. As a result of the extensive research into various methods of sample retention, even samples with relatively little difference in their physical or chemical structure can be separated. Chromatographic systems also range in size and complexity from a simple, gravity-fed packed column to a complex high pressure industrial-sized system with integrated components for sample introduction and fraction detection and collection. Often, chromatography is used in conjunction with another analytical method for determination of fraction purity and composition. For medical device instrumentation, chromatography is an invaluable technique that may find its application in a wide variety of ways such as protein purification and sample contamination detection.
Numerous journal articles and books have been written on the subject, as well as reviews (3) and a host of information published on the Internet (2,4–6). Several journals dedicated to chromatography and related fields also exist (Advances in Chromatography, Chromatographia, Biomedical Chromatography, Journal of Chromatography, Journal of Liquid Chromatography and Related Technologies, Journal of Planar Chromatography, Journal of Separation Science). This information is well accessible to anyone who has interest in pursuing the methods and techniques described in this chapter. No attempt has been made here to review all this material. For this reason, this chapter will not focus on the details and extensive literature, but on the

102 CHROMATOGRAPHY
Figure 1. Depiction of a basic chromatographic system with the major components: carrier or mobile phase, sample bolus, column containing the stationary phase, concentration or massbased detector, and the effluent. Also shown are the theoretical equilibrium plates, each width being one plate height.
basics and presenting what is generally possible with chromatography.
Chromatographic systems have a mobile phase and a stationary phase. The mobile phase is used to transport the samples through the stationary phase. The mobile phase is inert and does not interact with the sample or stationary phase. The stationary phase is unique for each separation because its purpose is to provide selective interaction with the samples. A simple chromatographic system is depicted in Fig. 1. Apart from the two fundamental required components just mentioned, a system must typically contain a sample injection port and detector in order to be useful as an analytical system. If it is to be used as a purification method, then it must also contain some method of recovering the fractions in the effluent.
The basic operating principle is that samples in the mixture, which interact to a higher degree with the stationary phase, travel slower and are retained longer in the system. This interaction, speed, and retention is relative to samples that interact to a lesser degree and so travel faster and are retained for a shorter duration. The spatial separation occurs in the mobile phase flow direction.
Chromatographic methods are grouped either into liquid or gas chromatography, based on the carrier phase and further identified by its particular method of sample retention. For example, in size-exclusion chromatography, the stationary phase is a bed of porous beads. The pores allow only samples smaller than a particular size to enter and then exit again. As a result of the pores tortuosity, the travel distance for these smaller samples is longer and thus increases the residence time relative to the larger samples. Some other methods include gas-adsorption, gas-liquid, capillary gas, liquid-adsorption, liquid-liquid, supercritical fluid, ion exchange, and affinity. In addition, the closely related fields of electrophoresis and FFF need to be mentioned as they typically complement chromatographic methods. In order to more fully understand the methods mentioned here, the basic theory will be mentioned first and then a more detailed description of the methods will follow.
GENERAL THEORY
The basic measurement in chromatography is the retention factor. This measurement is calculated from the measured retention times (t0, tA, tB, . . ., tn) in the chromatogram output, as shown in Fig. 2. The void time t0 is the elution time of an unretained sample. It is the time required to sweep one-column volume. All retention values
are calculated relative to this time. The retention factor for sample A, RA, is then
RA ¼ tA t t0
0
where tA is the sample residence time. The ability of the separation column to distinguish between two components is quantified in the selectivity term S
SAB ¼ RS
RA
.
For mixtures, the degree of separation between two components is termed the resolution RS. In most cases, the resolution is the most important factor because that is the purpose of performing chromatographic separations. It is calculated from the retention times t and the peak widths w for the two samples as
RS ¼ 2ðtB tAÞ
WB þ WA
The relative distance in the system at which a degree of equilibrium between the two phases occurs is referred to as a theoretical plate. Chromatographic systems usually have many theoretical plates. By dividing the length of the column L with the number of plates N gives the plate height, or height of an equivalent theoretical plate H. These numbers characterize the quality of the retention and can be determined from a chromatogram by assuming
response |
|
tB |
|
tA |
|
|
Sample A |
|
Detector |
|
|
t0 |
Sample B |
|
|
|
|
|
|
Void |
|
|
Time |
Figure 2. Chromatogram of separation of a two-component mixture. The retention times are measured from the injection time.
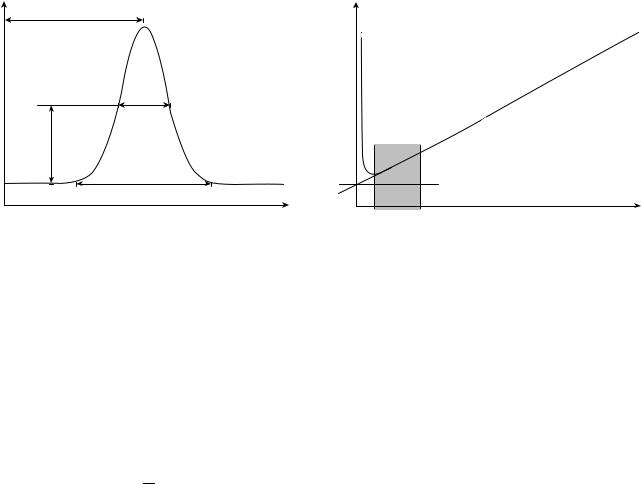
Detector response |
tR |
W1/2 |
Half height
W
Time
Figure 3. Chromatogram of single peak from response of massor concentration-based detector showing measurements for determining the number of theoretical plates.
that the peaks are Gaussian shaped, as shown in Fig. 3. It is noted that this method is for an ideal situation and that other methods are available for peaks of other shapes. The sample peak width is measured either at baseline w or at half-height, w1/2. In practice, it is more convenient and accurate to measure at half-height
!2
N ¼ 5:54 |
tR |
W1=2 |
and
H ¼ NL
Plate height is a summation of three effects, two of which are dependent on flow rate. This theory was proposed by van Deemter et al. (7). The first effect is due to equipment and users, such as column-packing and injection variabilities, and is not a function of the flow rate. This term can be minimized through careful design and manufacturing of the column. The use of automated sample handling also helps to reduce this effect. At low flow velocities u, molecular diffusion of the sample in the carrier dominates and peak broadening rises quickly. At higher flow velocities the sample plug broadens due to nonequilibrium effects such as eddy diffusion and multiple paths in the stationary phase. The van Deemter equation is
H ¼ H0 þ H1ðu 1Þ þ H2ðuÞ
This equation is easily graphed (Fig. 4) for a visual indication of the optimal flow rate. In practice, it is best to have the flow rate slightly higher than at the optimum to avoid the region of rapid plate height increase. Each of the terms is dependent on the type of chromatography used.
Now, the resolution of the separation can be put in terms of plate height or the equivalent number of theoretical plates,
RsAB |
|
p |
|
|
SAB 1 |
|
1 þ RB |
|
|
¼ 4 |
SAB |
RB |
|||||
|
|
|
N |
|
|
|
|
|
CHROMATOGRAPHY 103
Plate height |
Molecular diffusion |
|
(mobile phase) |
Nonequilibrium (stationary phase)
Equipment
Optimal range |
Flow velocity |
|
Figure 4. The van Deemter plot, based on the van Deemter equation for determining the optimal flow rate for a given chromatographic separation.
This form is useful for optimizing a separation based on the three groups in the equation. Resolution can be increased by increasing the number of theoretical plates, which can be accomplished by both increasing the column length and minimizing the plate height using the van Deemter plot. However, increasing the column length will also cause proportional band broadening, and so it is not always ideal. The second term involves adjusting the selectivity through column modifications such as changing either or both of the phases and the temperature. The temperature also plays an important role in the last term as well. By adjusting and tuning these parameters, nearly every difficult separation can be successfully resolved. Theoretically, choosing the proper stationary phase for the column and an appropriate mobile phase, any two materials can be separated. These phases are at the heart of chromatography.
TYPES OF CHROMATOGRAPHY AND RELATED TECHNOLOGIES
The mobile phase is either a gas, a liquid, or a supercritical fluid, whereas the stationary phases can be either a solid or a liquid. The types of chromatography are typically named by their phases or interaction process and are categorically divided by their stationary phase into either gas chromatography (GC) or liquid chromatography (LC). Another variation on LC is high performance liquid chromatography (HPLC), in which the carrier is driven by pressure. Some common subclasses of LC and GC are based on ionexchange, phase change, adsorption, size exclusion, partitioning, and absorption. Specific types and hybrid systems also exist that fall under each of these categories. Each of these complements the others to build a broad spectrum of types of chromatographic separation technique available (8–11).
Gas Chromatography (GC)
Gas chromatography makes use of a pressurized gas cylinder and a carrier gas, such as helium, to carry the solute
104 CHROMATOGRAPHY
through the column. GC can be used for both purification and analysis, when a detector is used in tandem. Common detectors used in GC are thermal conductivity and flame ionization detectors. Many more types of detectors exist, each with its advantages and disadvantages. Three types of GC that are among the more common methods are gas adsorption, gas-liquid, and capillary gas chromatography.
Gas Adsorption. Gas adsorption chromatography has a solid stationary phase packed bed. The samples selectively adsorb and desorb to the stationary phase, effectively increasing each sample’s retention time based on its isotherm. Some of the more common adsorbents used are silica, zeolite, and activated alumina. This method is the primary method for separating mixtures of gases.
Gas-Liquid. Separation in gas-liquid chromatography is based on the gaseous samples partitioning with a viscous liquid stationary phase. This liquid is supported in the column by coating a solid, most commonly diatomaceous earth. The solid support to the stationary phase liquid is inert to the samples. The sample retention time is governed by the rate at which it dissolves into and vaporizes out of the liquid. Thus, the relative partitioning of each of the samples in the liquid stationary phase is the basis of the separation.
Capillary Gas. In this method, the stationary phase is a capillary coated with a liquid (wall-coated open tubular) or a solid-coated capillary onto which the liquid is adsorbed (support-coated open tubular), as has been described in the previous two methods. Liquid or gum temperature stable polymers are used as the stationary phase. Most common polymers used are poly-ethylene glycol or poly-siloxanes. Also used are molecular sieves and alumina particles. Unlike the previous methods, the stationary phase has a small volume due to the capillary geometry and is thus limited to the amount of sample that can interact. However, because of the small column geometry, the partitioning or adsorption of the sample is relatively fast. The capillary is typically glass or fused silica coated with polyimide for support. The tubing (column) can be long and also be wound into tight areas for compactness and good temperature control. It is the most common gas chromatography analytical method.
Liquid Chromatography (LC) and High Performance Liquid
Chromatography (HPLC)
As the carrier phase in LC is a liquid, it is naturally more amenable for biological separations and analysis, such as the purification of proteins. However, it is also amenable to any sample dissolved in a liquid. In LC, the carrier is driven by gravity through the column. These columns, made of glass or plastic and sometimes disposable, are typically used for lab-scale preparative work. For analysis of samples, the carrier is pressurized for increased speed and sample resolution. This variation is termed high performance liquid chromatography or HPLC. These systems are much more complex and costly. The columns are made of steel to withstand high pressures and are reused a
number of times. Detectors are also placed inline with these columns for analysis, although HPLC is also used in preparative work as well (3,6,11–14).
Liquid Adsorption. Liquid adsorption, also termed liquid-solid chromatography, uses a solid stationary phase made of particles such as alumina or silica. In particular, this method is used in separating isomers. The retention is based on the adsorption/desorption kinetics of each sample onto the particles. Liquid adsorption is often found in largescale applications because the adsorbent beds are relatively inexpensive.
Liquid-Liquid. In liquid-liquid chromatography (LLC), also called partition chromatography, the stationary phase is a liquid-coated solid surface. This liquid is immiscible with the liquid solvent mobile phase. Retention is based on partitioning of the sample between the two phases. LLC can be accomplished in either normal phase or reverse phase. Normal phase has a nonpolar mobile phase and polar stationary phase. Reverse phase is the opposite of having a polar mobile phase and nonpolar stationary phase. It is used primarily in separating nonvolatile components of mixtures and is similar to a chemical extraction process.
Size Exclusion. This method was described briefly in the introduction. It is somewhat unique because the stationary phase is inert to the sample. The increased path length due to tortuous pores that exclude large samples causes an increased retention time for samples smaller than the cutoff size. It is also referred to as filtration, gel permeation, or molecular-sieve chromatography. This method is useful for protein separation and purification such as in antibody production and buffer exchange applications.
Supercritical Fluid. Unlike the other methods, supercritical fluid chromatography is characterized by its unique carrier fluid. Supercritical fluids used to carry the sample have very high viscosities and molecular diffusivities compared with liquids but with densities on the same order. One type of supercritical fluid used is a mixture of carbon dioxide and modifiers. Implementation of this technique is difficult because of the high temperature and pressures to reach the supercritical fluid state.
Ion-Exchange. Ion-exchange chromatography is commonly used in the purification of biological materials, such as amino acids and proteins, and also ions in solution. This method is capable of quantifying samples in the ppb to ppm concentration range. The stationary phase is an ionexchange resin that is either cationic or anionic. Charged atoms or molecules in the liquid phase sample bind to the stationary phase as they are passed through the column. The sample is released by adjusting the carrier pH or ionic strength. Separation by this method is highly selective and especially useful for anions in which separations are typically slow. The resins are typically high capacity and inexpensive.
Affinity. Affinity chromatography has a stationary phase that is highly selective to one particular sample.
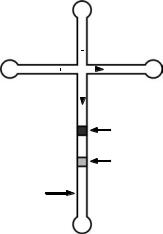
Unlike other chromatographic methods, the sample is highly bound to the stationary phase until the carrier solution is changed and the sample released. To accomplish this selective release, the stationary phase is engineered using an inert affinity matrix, such as agarose or cellulose derivative, and infused with ligand molecules that are design to bind only the sample of choice. Immunologic interactions of specific antibody-antigen pairs are particularly useful because of the high specificity that can be obtained and the reversibility of the binding event. The addition of a high salt concentration or low pH to the stationary phase reverses the selectivity, similar to ionexchange chromatography, and allows the release of the sample after the other components of the mixture have been washed away. Care must be taken to ensure that impurities do not foul the matrix. Some preprocessing is typically accomplished prior to the separation to remove the potential fouling components. This method is used often with biological samples.
Electrophoresis
Electrophoresis is a separation method using the transport of electrically charged compounds in a conductive liquid environment under the influence of an electric field (15,16). Positively charged molecules migrate toward a negative electrode, and negatively charged molecules migrate toward a positive electrode. It is regularly applied in analytical chemistry to determine the constituent molecules of a compound. It is also widely used in medical diagnostics and other biological areas to determine molecules within biological samples, such as protein and DNA. From the various modes of electrophoresis, capillary electrophoresis (CE) is the most widely used separation method used in a modern analytical laboratory (17). High separation speed, excellent resolution power, and low consumption of buffer and sample are some of the advantages. Typically, samples are injected into a capillary tube with diameters ranging between 25 and 100 mm, and an electrical field is applied along the capillary tube to separate compounds based on the differences in charge to mass ratio. Negatively ionized surface silanol group of the capillary creates an electrical double layer at the solid/liquid interface to preserve electroneutrality, and this mobile layer is pulled toward the negatively charged electrode when an electric field is applied. These ion layers drag the bulk buffer solution, causing an electro-osmotic flow. Compared with HPLC, which has a parabolic flow profile due to the laminar flow inside the channel, the flow profile is flat in the electro-osmotic flow, which helps the detection peak to be very sharp, increasing its sensitivity. Laser-induced fluorescence detection is the most widely used method for detecting the separated molecules (18). Over 100 review articles exist covering capillary electrophoresis, and Beale wrote an excellent review categorizing these articles (19).
Development in microfabrication technologies and the lab-on-a-chip concept in the early 1990s further expanded the role of this powerful analysis technique (20–22). Smaller sample injection into a microchannel and higher electric field result in short analysis time with excellent resolution. Applying higher electric field is possible due to the high
CHROMATOGRAPHY 105
surface-to-volume ratio of a microchannel that can dissipate the heat produced during electrophoresis faster. Automated sample injection and capability to perform the separation on arrays of microchannel in conjunction with the short analysis time enables high throughput analyses. Low manufacturing cost due to the batch fabrication capability of the microchips is another advantage over conventional separation technologies. Fig. 5 shows a typical channel configuration for a capillary electrophoresis microchip. High voltages are applied between reservoir 1 and 2 so that the sample in reservoir 1 fills the injection channel. Once the injection channel is filled, the high voltages are switched to reservoir 3 and 4, and the sample plug in the cross section gets injected into the separation channel. As the sample plug moves down the separation channel, separation occurs depending on the charge to mass ratio of the compounds being analyzed. In fluorescence detection, detection typically occurs at the end of the separation channel by illuminating the fluorescence-tagged sample with laser or UV light, followed by light detection using a photomultiplier tube (PMT).
Typical channels are several tens to hundreds of microns wide, a couple tens of microns deep, and the separation channel lengths are in the centimeter scale. Electric fields applied to these channels range in kV/cm scale. This high electric field and small sample size enables separation within several seconds compared with tens of minutes required in conventional chromatography, which can be of great benefit when monitoring time-dependent reactions, such as conducting an enzyme kinetic study (23). This system also enables automatic sample injection because voltages can be simply switched between reservoirs to inject samples into the separation channels without the need for manual sample loading, which further reduces the time associated with sample analyses. Several other sample injection schemes have been also studied. Instead of using a simple cross-channel injector, twin-T injectors can be used to further control the sample plug size (24). Electrical biasing of the different reservoirs such as
|
3 |
||
Injection |
|
|
|
channel |
|
|
|
1 |
|
|
2 |
|
|
||
|
|
|
|
Separation |
Sample1 |
|
channel |
||
|
||
|
Sample1 |
Detection site
4
Figure 5. Typical channel configuration for a capillary electrophoresis microchip including loading or injection channel and separation channel.

106 CHROMATOGRAPHY
Figure 6. CE microchip fabricated in borosilicate glass. Serpentine separation channel can be observed.
pinch injection can reduce band broadening of the sample plugs caused by leakage at the intersection of the injection channel (25). Fig. 6 shows a CE microchip made in borosilicate glass. Serpentine separation channel was created to have a longer separationchannel withinacompactgeometry.
The most common material used for the microchip is glass. Some of the earliest capillary electrophoresis microchips were fabricated in glass due to the good optical properties, a surface that enables electro-osmotic flow, and well-developed microfabrication techniques. To further reduce the microchip fabrication cost, polymer materials have been used due to the low material cost and easy massfabrication processes. Injection molding or hot embossing of plastics and polymer casting of polydimethylsiloxane (PDMS) are some of the methods used (26–28).
The application of this technology has been expanded from simple chemical analysis to biological applications, such as DNA sequencing, immunoassay, and biological particle separation (viruses, bacteria, and eukaryotic cells) (22,29–31). DNA sequencing on microchips was first reported by Mathies in 1995 (32). Single base resolution reached 150–200 bases in 10–15 min. Some of the advantages on top of the excellent resolution and short analysis time are the capability for high throughput. The compact size of the separation channels enable a large number of channels to be placed close together, which also facilitates fast detection using optical imaging or scanning lenses. To pack even more channels into a small area, a 6 inch circular glass plate carrying 96 radical channels conversing at the center of the chip was also developed (33). Detection occurred using a spinning confocal system. Separation of antigen and antibody from the corresponding anti- body-antigen complex can be separated using microchip CE for immunoassays (34). Automatic sample injection capability can possibly eliminate the need of conventional robotic sample injection, which is commonly used in life science laboratories. More complex operations, by using electro-osmotic flow in conjunction with other operations such as lysing and concentration, have been demonstrated to show transport and analysis of biological particles (35–37). Dual injection has been also used where sample and reagents can be mixed on-column and analyzed to provide information about reaction kinetics, to
perform on-column derivatization for improved separation and termination, and to develop methods for simultaneous analysis of anionic and cationic compounds (38). Electro-osmotic flow in microchannels has been used in numerous applications to transport and mix fluid and particles but is beyond the subject of this chapter (39,40).
Electrophoresis with Other Separation/Detection Methods
One of the advantages of capillary electrophoresis is that this technique is easy to combine with different separation and detection methods to provide even more versatile, powerful, and efficient analysis tools. Isoelectric focusing (IEF) is a separation technique to resolve amphoteric molecules based on their isoelectric points (pI) (41). Isoelectric point is the pH at which a molecule carries no net electric charge. In capillary isoelectric focusing (CIEF), the capillary is first filled with a mixture of ampholytes and samples (42). When an electric field is applied to the capillary, a pH gradient is formed inside the capillary and the sample molecules migrate and stop at a position where the pH equals the pI of the sample molecules due to the loss of their net charges. In a one-step process, the entire capillary is illuminated to obtain images of the separation. In a two-step process, the separated samples are mobilized to the detection point using chemical, hydrodynamic, or electro-osmotic flow mobilization to simplify the detection equipments. When analyzing mixture of peptides in a microchip-based CIEF, focusing time of less than 30 seconds and total analysis time as short as 5 minutes is possible. As a result of the high resolving power, this method is most commonly used for studying peptides, proteins, recombinant products, cell lysates, and other complex mixtures (43,44).
Although capillary electrophoresis can provide excellent resolution, it is challenging to identify unknown substances. Mass spectrometry is a technique used for separating ions by their mass to charge ratios that enables identification of compounds by the mass of one or more elements in the compounds and enables determination of isotopic composition of one or more elements in the compound. By coupling capillary electrophoresis with mass spectrometry, direct identification of analytes by molecular mass, selectivity enhancement, and insight into the molecular structures are possible (43,44). The most prominent application of this combination is in proteomics (45). Interfacing these two techniques is of great importance because mass spectrometry requires ionized gas as samples whereas the output from a CE system is fluid (46). Electrospray ionization (ESI) and matrix-assisted laser desorption/ionization (MALDI) are some of the most widely used ionization methods. ESI, first developed in the 1980s, is the softest ionization technique currently available. It transforms ions in solution into ions in gas phase based on the electrostatic effects in solutions. An electric potential applied to an electrospray tip breaks the solution containing mixture of samples and solvents into small charged droplets. The shrinkage of the charged droplets by solvent evaporation further disintegrates the drops and forms gas-phase ions. MALDI uses laser beams to ionize samples located inside a crystallized bimolecular matrix
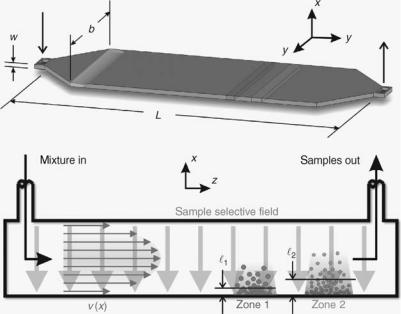
that is used to protect the sample from being destroyed by direct laser beam. For microchip-based capillary electrophoresis systems, efforts have been focused on developing on-chip electrospray ionization techniques so that coupling to MS systems are efficient (47). The ultimate goal of such a coupled system is to use the microchip for fast and convenient sample preparation followed by online sample introduction for MS analysis. Beyond proteomics, the CE-ESI-MS combination has been widely used for drug analysis, food analysis, achiral and chiral solutes analysis, glycoscreening, and metabolic disorder screening (48–52).
Other detection methods used include electrochemical detection (53), electrochemiluminescence, nuclear magnetic resonance (NMR), ultraviolet resonance Raman spectroscopy (54,55).
Field-Flow Fractionation
Another technique similar in many ways and complementary to chromatography is field-flow fractionation (FFF). It is relatively young compared with chromatography, proposed by Giddings in 1968. This technique is always performed in an open channel (no packing or coatings) that is usually, but not restricted to, a wide, flat geometry with the breadth to height ratio being greater than 100 (Fig. 7). The purpose of this geometry is to take advantage of the laminar velocity parabolic flow profile of the carrier while minimizing secondary dispersion effects from the sidewalls. Circular channels can also be used in this way but are difficult to implement a uniform field in. Just as in chromatography, the samples spatially separated along the length of the channel are eluted discretely at the outlet, if enough column length and separating power are provided. For this reason, chromatographic principles and basic theory also apply to FFF. For example, FFF system retentions are often characterized by the number of theoretical plates and the van Deemter theory and the separa-
CHROMATOGRAPHY 107
tions are characterized by resolution. However, some distinct differences exist that demonstrate the unique and complementary characteristics of FFF.
The first difference between FFF and chromatography is that a force field is applied to affect the samples instead of a stationary phase. The second difference is that the field is applied normal to the flow and separation direction. In chromatography, this field always acts opposite to the direction of the separation. The third difference is that, in most simple FFF systems, a direct mathematical relationship exists between the field and sample elution time.
The mechanism of retention and separation in FFF systems is based on compartmentalizing the various samples in the mixture to velocity zones in the parabolic flow profile of the carrier. The samples are selectively perturbed using a field applied normal to the carrier flow that are then concentrated at the accumulation wall. Normal diffusion opposes this movement until an equilibrium condition is established. Each sample will form a layer thickness based on its degree of perturbation. The sample specific velocity is then obtained from the first moment of the concentration and velocity profiles. The exact mathematical relationship among the variables in each of these steps allows for the determination of specific sample properties based solely on the elution time. A simple concentration or mass-based detector is sufficient without the need for calibration as in chromatography. In practice, this type of analysis is complicated by other factors leading to the need for calibration or more complicated detectors.
Any type of field or combination of fields can, in theory, be used to drive the separation. Some of the common fields used are sedimentary, flow, thermal, and electric. Some less common fields include magnetic, acoustic, dielectric, and others.
Recent advancements in FFF have included miniaturization of FFF channels. Miniaturization not only reduces sample and carrier volume requirements but also enhances
Figure 7. Top: Representation of a typical FFF channel. Bottom: Cross-sectional slice of channel in the center along the length and showing the operational principles of a separation in FFF. The required components for retention are a parabolic velocity profile and sample selective field.
108 CHROMATOGRAPHY
retention and separation in some cases. Some of the systems that benefit through field enhancement from reducing the scale are thermal, electric, magnetic, and dielectrophoretic. In addition, microscale and nanoscale fabrication technologies have also made possible the implementation of systems that were previously impossible, difficult, or unreasonable to try, such as acoustic, magnetic, and dielectrophoretic FFF, as well as combinations of these systems.
Several microscale systems have been successfully implemented: micro-electric FFF (56–60); micro-thermal FFF (61–66); and AcFFF by Edwards and Frazier (67). Gale also integrated an electrical impedance detector within the channel to minimize the plate height due to extracolumn volumes in the detector and between the column and detector (56). Both Gale and Edwards also developed sample injection methods to minimize plate height further (56,61).
Systems manufactured using microelectricalmechanical system (MEMS) technologies are not only suitable for creating an inexpensive, disposable analysis device, but also for integrating with other methods such as chromatography, sensors, fluid handling devices, and actuators to create a total analysis system, or lab-on-a-chip. FFF can be used as a sample preparation tool, analytical device, or both in these systems.
CONCLUSION
Chromatography and the closely related fields of FFF and electrophoresis have proven to be valuable methods over the last century for separation, purification, and analysis. As a result of the wide variety and combinations of phases used in chromatography and fields applied in FFF, the number of types of samples that have been or can be retained and separated in these systems appears to be endless. Further advances in column, carrier, and detector technology, such as miniaturization, will continue to push the limits outward and make available faster and higher quality separations. In turn, researchers and industries in fields such as chemical engineering, bioengineering, chemistry, and pharmaceutics that rely on these techniques will also advance.
BIBLIOGRAPHY
1.Tswett M. Physical chemical studies on chlorophyll adsoptions. Berichte der Deutschen botanischen Gesellschaft 1906;24: 316–323.
2.Lesney MS. A brief history of ‘‘color writing.’’ Today’s Chemist at Work 1998;7(8):67–72.
3.Dorsey JG, et al. Liquid chromatography: Theory and methodology. Analyt Chem 1998;70(12):591R–644R.
4.Carrier R, Yip K. Intro to Chromatography. Available http:// www.rpi.edu/dept/chem-eng/Biotech-Environ/CHROMO/ chromintro.html.
5.Hardy JK. Analyt Chem. Available http://ull.chemistry.vakron. edu/analytical/.
6.Kazakevich Y, McNair H. Basic liquid chromatography. Available http://hplc.chem.shu.edu/NEW/HPLC_Book/.
7.van Deemter J, et al. Longitudinal diffusion and resistance to mass transfer as causes of nonideality in chromatography. Chem Eng Sci 1956;5:271–289.
8.Brede C, Pedersen-Bjergaard S. State-of-the art of selective detection and identification of I , Br , Cl , and F-containing
compounds in gas chromatography and liquid chromatography. J Chromatogr A 2004;1050(1):45.
9.Gonzalez FR. Application of capillary gas chromatography to studies on solvation thermodynamics. J Chromatog A 2004; 1037(1-2):233.
10.Roubani-Kalantzopoulou F. Determination of isotherms by gas-solid chromatography: Applications. J Chromatogr A 2004;1037(1-2):191.
11.He L, Beesley TE. Applications of enantiomeric gas chromatography: A review. J Liquid Chromatogr Related Technol 2005; 28(7-8):1075.
12.Abraham MH, et al. Hydrogen bonding. 42. Characterization of reversed-phase high-performance liquid chromatographic C-18 stationary phases. J Phys Organ Chem 1997;10(5):358–368.
13.Sherma J. High-performance liquid chromatography/mass spectrometry analysis of botanical medicines and dietary supplements: A review. J AOAC Int 2003;86(5):873.
14.Petrovic M, et al. Liquid chromatography-tandem mass spectrometry for the analysis of pharmaceutical residues in environmental samples: A review. J Chromatogr A 2005;1067(1-2):1.
15.Kuhn R. Hoffstetter-Kuhn S. Capillary Electrophoresis: Principles and Practice. Berlin, Germany: Springer-Verlag; 1993.
16.Baker DR. Capillary Electrophoresis. New York: Wiley-Inter- science; 1995.
17.Horvath C, Nikelly JG. Capillary Electrophoresis and Chromatography. Washington, DC: American Chemical Society; 1990.
18.Nouadje G, et al. Capillary electrophoresis with laser-induced fluorescence detecion: Optical design and applications. Progress in HPLC-HPCE 1997;5:49–72.
19.Beale SC. Capillary electrophoresis. Analyt Chem 1998;70:279R– 300R.
20.Effenhauser CS. Integrated chip-based microcolumn separation systems. Topics Curr Chem 1998;194:51–81.
21.Regnier FE, et al. Chromatography and electrophoresis on chips: critical elements of future integrated, microfluidic analytical systems for life science. Trends Biotechnol 1999;17(3): 101–106.
22.Dolnik V, et al. Capillary electrophoresis on microchip. Electrophoresis 2000;21:41–54.
23.Starkey DE, et al. A fluorogenic assay for b-glucuronidase using microchip-based capillary electrophoresis. J Chromatogr B 2001;762:33–41.
24.Effenhauser CS, et al. Glass chips for high-speed capillary electrophoresis separations with submicrometer plate heights. Analyt Chem 1993;65:2637–2642.
25.Jacobson SC, et al. Effects of injection schemes and column geometry on the performance of microchip electrophoresis devices. Analyt Chem 1994;66:1107–1113.
26.Effenhauser CS, et al. Integrated chip-based capillary electrophoresis. Electrophoresis 1997;18:2203–2213.
27.Martynova L, et al. Fabrication of plastic microfluidic channels by imprinting methods. Analyt Chem 1997;69:4783–4789.
28.McCormick RM, et al. Microchannel electrophoretic separations of DNA in injection-molded plastic substrates. Analyt Chem 1997;69:2626–2630.
29.Colyer CL, et al. Clinical potential of microchip capillary electrophoresis systems. Electrophoresis 1997;18:1733–1741.
30.Effenhauser CS, et al. Integrated capillary electrophoresis on flexible silicone microdevices: Analysis of DNA restriction fragments and detection of single DNA molecules on microchips. Analyt Chem 1997;69:3451–3457.
31.Carrilho E. DNA sequencing by capillary array electrophoresis and microfabricated array systems. Electrophoresis 2000;21: 55–65.
32.Woolley AT, Mathies RA. Ultra-high-speed DNA sequencing using capillary electrophoresis chips. Analyt Chem 1995;67: 3676–4086.