
Neutron Scattering in Biology - Fitter Gutberlet and Katsaras
.pdf418J. Fitter
27.J. Heberle, J. Fitter, H.J. Sass, G. B¨uldt, Biophys. Chem. 85 229, (2000)
28.T. Lazaridis, I. Lee, M. Karplus, Protein Sci. 6, 2589 (1997)
29.P. Zavodszky, J. Kardos, F. Svingor, G. Petsko, Proc. Natl Acad. Sci. USA 95, 7406 (1998)
30.J. Fitter, J. Heberle, Biophys. J. 79, 1629 (2000)
31.M. Tehei, D. Madern, C. Pfister, G. Zaccai, Proc. Natl Acad. Sci. USA 98, 14356 (2001)
32.E. Haustein, P. Schwille, Methods 29, 153 (2003)
33. F.X. Schmidt, in: Protein Structure: A Practical Approach, ed. by T.E. Creighton (IRL Press, Oxford, 1989), pp. 299–321
34.C.N. Pace, J.M. Scholz, in: Protein Structure: A Practical Approach , ed. by T.E. Creighton (IRL Press, Oxford, 1989), pp. 261–289
35.K. Gast, G. Damaschun, R. Misselwitz, D. Zirwer, Eur. Biophys. J. 21, 357 (1992)
36.I. Nesmelova, V.D. Skira, V.D. Fedotov, Biopolymers 63, 132 (2002)
37.C. Zander, J. Enderlein, R.A. Keller, Single Molecule Detection in Solution
(Wiley-VCH, Berlin, 2002)
38.D. Russo, J. Perez, J.M. Zanotti, M. Desmadril, D. Durand, Biophys. J. 83, 2792 (2002)
39.M. Bee, in: Quasi-Elastic Neutron Scattering (Hilger, Bristol (UK), 1988)
40.V. Receveur, P. Calmettes, J. Smith, M. Desmadril, G. Coddens, D. Durand, Proteins. 28, 380 (1997)
41.J. Perez, J.M. Zanotti, D. Durand, Biophys. J. 77, 454 (1999)
42.Z. Bu, D.A. Neumann, S.H. Lee, C.M. Brown, D.M. Engelmann, C.C. Han, J. Mol. Biol. 301, 525 (2000)
43.M.C. Bellissent-Funel, J.M. Zanotti, S.H. Chen, Farraday Discuss. 103, 281 (1996)
44.R.E. Lechner, N.A. Dencher, J. Fitter, T. Dippel, Solid Sate Ionics 70/71, 296 (1994)
45.J. Fitter, R.E. Lechner, N.A. Dencher, Phys. Chem. B 103, 8036 (1999)
46.M. Tarek, D.J. Tobias, J. Am. Chem. Soc. 121, 9740 (1999)
47.C. Bon, A.J. Dianoux, M. Ferrand, M.S. Lehmann, Biophys. J. 83, 1578 (2002)
48.J.A. Hayward, J.L. Finney, R.M. Daniel, J.C. Smith, Biophys. J. 85, 679 (2003)
49.J. Fitter, R. Herrmann, T. Hauss, R.E. Lechner, N.A. Dencher, Physica B 301, 1 (2001)
50.Z. Bu, J. Cook, D. Callaway, J. Mol. Biol. 312, 865 (2001)
51.W. Doster, R. Gebhardt, Chem. Phys. 292, 383 (2003)
52.J.E. Nielsen, T.V. Borchert, Biochim. Biophys. Acta 1543, 253 (2000)
53.J. Fitter, R. Herrmann, N.A. Dencher, A. Blume, T. Hauss, Biochemistry 40, 10723 (2001)
54.D. Georlette, V. Blaise, T. Collins, S. D’Amico et al., FEMS Microbiol. Rev. 28, 25 (2004)
55.F. Volino, A.J. Dianoux, Mol. Phys. 41, 271 (1980)
56.J.N. Onuchic, Z. Luthey-Schulten, P.G. Wolynes, Annu. Rev. Phys. Chem. 48, 545 (1997)
57.J. Fitter, Chem. Phys. 292, 405 (2003)
58.A. Fersht, in: Structure and Mechanism in Proteins Science (W.H. Freeman and Company, New York, 1999)
59.R. Becker, in: Theorie der W¨arme (Springer Verlag, Berlin, 1985)
60.T.P. Creamer, Proteins 40, 443 (2000)

18
Relating Protein Dynamics to Function and Structure: The Purple Membrane
U. Lehnert, M. Weik
18.1 Introduction
Biological macromolecules have a highly complex structure and this is reflected in their dynamical behavior. The dynamical spectrum covers a time domain of several orders of magnitude from 10−14 to 1 s and longer [1]. High frequency motions such as vibrations of bonded atoms are localized over a few atoms. Their energy varies from 35 to about 350 meV and the amplitudes are in a range from 0.01 to 0.15 ˚A. The low frequency modes such as vibrations of globular regions, side-chain rotations at the surface, or torsional motions may involve more sizeable masses and can be considered as rigid body motions. They can be thermally excited at 300 K in an energy range from 0 to 25 meV. Neutron scattering probes such kind of rigid body motions. The investigated energy range ( eV–meV) corresponding to the nano-second to pico-second time-range, reflects motions of the atomic groups to which the H-atom is associated [2, 3].
Fluctuations of local or global character are believed to play an important role in protein function [4]. A description of protein motions has been suggested by Frauenfelder and coworkers [5, 6], in which the potential energy is described by a multidimensional energy landscape as a function of conformational coordinates. A protein can assume a large number of nearly isoenergetic conformations with only small structural di erences. Each of the various protein conformations, represented by a valley, is divided into several conformational substates. Each conformational substate is organized in a hierarchy of a number of tiers. At low temperature, the protein atoms vibrate harmonically within their substate. As temperature increases, su cient energy is provided to cross from one well to another, which gives rise to di usive, nonvibrational dynamics. These motions can be investigated by quasielastic neutron scattering. Molecular dynamics simulations of myoglobin have shown that these di usive motions as seen in neutron scattering experiments result from rigidbody motions of the protein side-chains attached to the protein backbone [7]. A model has been developed based on a hydrated powder of C-phycocyanin,

420 U. Lehnert et al.
which decomposes protein dynamics into three components: global motions of the residues, side-chain rigid-body motions and internal deformation [8, 9]. It has been demonstrated that the global motions of the residues correspond to vibrations in local energy minima and jumps between minima, whereas the rigid-body side-chain motions mainly describe the nonharmonic component.
18.1.1 Elastic Incoherent Neutron Scattering
Harmonic and nonharmonic protein motions can be explored by elastic incoherent neutron scattering (for a review see [3]). We recall that in the Gaussian approximation (for further information see contributions by Lechner et al., Part I and Doster, this volume) the scattering function can be described as
Sin(Q, 0) = exp . (18.1)
Two factors determine, which motions can be observed in an elastic scattering experiment:
–The accessible Q-range. The wave-vector Q is proportional to the inverse of a distance. The higher the studied Q-value, the smaller the amplitudes of the observed motions.
–The energy resolution of the instrument. The better the energy resolution, the lower the frequencies which can be investigated, i.e., slower motions become accessible on the spectrometer.
If only the elastic component is studied, all motions in the time interval corresponding to the energy resolution contribute to the scattering, but are not resolved into di erent correlation times or frequencies. Only motions faster than the corresponding time limit are observed.
Mean square amplitudes, <u2>, are accessible as a function of temperature from the intensity of elastically scattered neutrons. These <u2> correspond to the full extend of the motion which has been swept out by the atom during a short time t. At low temperature, where only harmonic vibrations are present, the <u2> show a linear increase with temperature. A break in the increase of the mean square amplitude vs. temperature signals the onset of nonharmonic motions. This feature is termed a dynamical transition and has been observed in neutron scattering for hydrated protein powders and membranes. A first transition arises typically around 200 K and has been interpreted with several physical models, which will be detailed further in Sect. 18.2.2.
In the following sections we will elucidate how dynamics investigated by incoherent neutron scattering can be related to functional and structural characteristics of the biological system under study. We will focus on dynamics extracted from the elastic scattering intensity. In particular, we will highlight the membrane protein bacteriorhodopsin and exemplify the relationship between protein structure, dynamics, and hydration on one hand and protein function and activity on the other.
18 Relating Protein Dynamics to Function and Structure |
421 |
18.2 Methods of Investigation
18.2.1 Elastic Incoherent Neutron Scattering on Powder Samples
Protein dynamics was first studied in powder samples. Powder samples consist of dried proteins or proteins in various hydration states. In general, hydration (h) varies from 0 to 0.5 g of solvent per g of protein (g g−1). At a hydration value of h = 0.4 g g−1 a smaller protein is completely covered by one hydration shell and is in an active state [10]. The dynamics of powder samples can be investigated over a large temperature range. Owing to the absence of crystalline ice formation, there is no need to employ a cryoprotectant for studies at low temperatures as there is for liquid samples. Myoglobin is a widely studied system and a dynamical transition of Fe motions has first been observed by M¨ossbauer spectroscopy [11]. Later, neutron scattering revealed a dynamical transition for global motions at 180 K (Fig. 18.1) [12]. Further, the e ects of trehalose on myoglobin dynamics have been studied [13]. Trehalose is a disaccharide, which can protect biomolecules under extreme conditions (high temperature and low hydration) against dehydration and denaturation. When myoglobin becomes coated in trehalose it behaves like a harmonic solid up to room temperature. The dynamical transition formerly observed, is absent (Fig. 18.1). Moreover, it has been reported that characteristic motions as observed by M¨ossbauer, optical absorption spectroscopy [14] and obtained from MD simulations [15] are hindered. Therefore, it has been concluded that trehalose traps the protein in a conformational substate, inhibiting larger nonharmonic motions, which might lead to protein unfolding.
18.2.2 Models for Describing Thermal Protein Dynamics
Several models have been developed to describe protein motions. We focus here on models aiming to describe motions measured in energy resolved elastic incoherent neutron scattering.
A double-well model has been applied to explain the dynamical behavior of myoglobin (inset Fig. 18.1) [12]. At temperatures below the dynamical transition (part A of triangles in Fig. 18.1), the atoms are trapped in a harmonic well. The dynamical transition was observed at 180 K, which was interpreted as the onset of jumps of hydrogen atoms between states of di erent energy (part B of triangles in Fig. 18.1). These nonharmonic motions were modeled by a two site-jump di usion, where the sites are at a distance d with a free energy di erence ∆G. This model allows to extract parameters like jump distance d, enthalpy ∆H and entropy ∆S/R di erence from the mean square amplitude vs. temperature data.
The concept of force constants has been introduced by Zaccai and coworkers [16, 17] in order to quantify the molecular resilience of a protein structure. From the slope of the <u2> values with respect to the temperature,
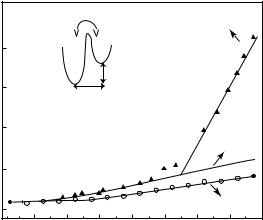
18 Relating Protein Dynamics to Function and Structure |
423 |
molecule. They can be used for comparison of di erent systems having similar transition temperatures. In this model, the dynamical transition temperature observed in the <u2> vs. temperature plot corresponds to the system gaining su cient energy to explore the upper well. The dynamical transition has been defined as the temperature where 10% of the atomic population is located in the outer potential of higher energy and displays larger amplitude motions [17].
18.2.3 H/D Labeling Techniques
As described in various chapters in this book, neutrons discriminate between di erent isotopes of an element leading to a di erent scattering cross-section for hydrogen than for deuterium. This particular specificity is not only employed in di raction experiments to localize hydrogen atoms or to minimize the solvent contribution to a scattering spectrum. Owing to the fact that the incoherent scattering cross-section of a hydrogen atom exceeds by far the one of deuterium and of other atoms in biological samples, a specific H/D labeling allows the study of local dynamics in the protein part where the hydrogens have been preserved while the deuterated part is masked. For neutron dynamics studies, only a few examples on specifically labeled samples exist.
For instance, a study focussing on hydration water used deuterated phycocyanin [18]. Another example deals with specifically labeled purple membranes [19]. In the latter case, only two types of amino acids have been labeled to investigate local core motions of the protein. This example will be presented in further detail in Sect. 18.3.3. An often discussed question is whether or not deuterium labeling alters the “real” motions of the system under study. One could argue that as neutron scattering experiments are sensitive to motions of the amino acid side-chains, deuteration could alter the dynamical spectrum. However, a typical contribution to the scattering spectrum is related to CH2 or CH3 groups and deuteration would only cause a minor change in mass. Moreover, a comparison of <u2> of fully deuterated PM with a natural abundance PM sample showed similar results [19]. However, to date not enough data are available for definitely excluding that deuterium labeling does not a ect the dynamics probed by neutron scattering and care should be taken when conceptualizing a project.
A facility for partial or full deuteration of biological molecules, such as proteins, nucleic acids and lipids has been set up by the Institut Laue-Langevin (ILL) and the EMBL Outstation in Grenoble. It aims to support the development and improvement of labeling techniques for biological systems and provides a user service for the production of deuterated samples for neutron scattering and NMR experiments (http://www.ill.fr/deuteration).
424 U. Lehnert et al.
18.3 Relating Thermal Motions in Purple Membranes to Structural and Functional Characteristics
of Bacteriorhodopsin
In the following we will present dynamical studies of di erent powder samples. In particular, we have chosen to highlight the relation between dynamics, structure and function of a biological system on the example of bacteriorhodopsin (BR), and the purple membrane (PM).
18.3.1 Thermal Motions in Bacteriorhodopsin and the Purple
Membrane
Purple membranes from halophilic Archaea display a 2D-crystalline arrangement in their native state and are composed of lipids and of only one type of protein, the light-driven proton pump bacteriorhodopsin [20]. Absorption of a photon by the chromophore retinal in BR triggers a cyclic series of ground and intermediate states (photocycle), namely bR, J, K, L, M, N, O, and bR [21,22]. As a result, one proton per photocycle is transferred from the cytoplasmic (CP) to the extracellular (EC) part of the membrane. The photocycle as well as the proton pump activity and their dependence on hydration and temperature have been thoroughly studied. Therefore, the relationship between structure – BR activity and protein dynamics can be explored on this system. BR and PM dynamics can be studied at several levels, each associated with interesting biological properties:
–Dynamical properties of the entire membrane as a function of temperature and hydration.
–Local motions by combining the powerful method of H/2H labeling and neutron scattering.
–The influence of the lipid environment on protein motions.
A protein is only active if it adopts its particular three-dimensional structure and if all internal motions attached to its function can be accomplished. Based on this, Zaccai [23] has suggested a hypothesis that the close packing of PM in a cooled or dry state would inhibit the motions necessary to complete the BR photocycle and the proton pumping across the membrane. A first set of incoherent neutron experiments, performed on PM in two extreme hydration states (dry: 0% relative humidity (r.h.), hydrated: 93% r.h), substantiated this hypothesis [24]. The investigation of mean square amplitudes, <u2>, as a function of temperature by elastic incoherent neutron scattering has revealed a transition around 230–250 K between harmonic and nonharmonic motions only for the hydrated PM sample (Fig. 18.2a, [24,25]). Below this temperature the hydrated sample, following a harmonic behavior, is not active, whereas above the transition temperature nonharmonic di usive motions appear and the BR photocycle can be completed. In contrast, the dry PM sample exhibits
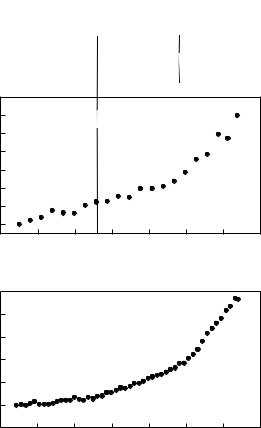
426 U. Lehnert et al.
di usive thermal motions, is required to ensure the functioning of BR. Complementary studies of the elastic incoherent scattering for smaller scattering vectors have demonstrated a dynamical transition at about 130–150 K, which is present for both the dry and the hydrated membrane (Fig. 18.2b [19, 28]). This has led to a division of PM dynamics into two di erent populations of motions: “high” amplitude motions exhibiting smaller frequency (maximum
2 |
˚2 |
at 300 K for the hydrated PM sample), and “small” ampli- |
||
<u |
> of 2 A |
|||
|
|
2 |
˚2 |
at 300 K for |
tude motions at a higher frequency (maximum <u |
> of 0.5 A |
the hydrated PM sample). The two dynamical transitions can be compared with the ability of BR to proceed in its photocycle (Fig. 18.2). The dynamical transition at 130 K (Fig. 18.2b) might be correlated with the ability of BR to convert from the K into the L intermediate. Large-scale structural changes that accompany the transition from an early to a late M state (i.e., from M1 to M2) correlate with the dynamical transition at 240 K (Fig. 18.2a and b).
A di erent approach using quasielastic incoherent neutron scattering on oriented PM samples has yielded information about the di usive motions of di erent spatial extent and with di erent correlation times in the nano-second to picosecond time scale [29]. This technique has allowed characterization of the main components of di usive motions such as three-site jump di u- sion of methyl group rotations and two-site jump di usion [30]. The onset of quasi-elastic scattering has been found around 200 K with a particular strong increase of this component around 260 K [31]. Moreover, the di erent categories of di usive motion have been found to depend on the hydration state of PM [29], supporting the hypothesis that a decrease in hydration results in a solidification in internal molecular flexibility of the protein structure. It has been concluded that the observed di usive motions are essential for BR photocycle activity.
18.3.2 Hydration Dependence of Thermal Motions
The hydration dependence of the thermal motions has been investigated further in a broad temperature range [25, 30]. “Fast” and “slower” motions as defined by the correlation time from quasielastic measurements have shown to be influenced di erently by hydration. In general, the slower local di usive motions become much more pronounced at a high hydration state, which led to the suggestion that they correspond to motions of groups close to the surface of the membrane [30]. The behavior of the <u2> values, as a function of temperature and hydration degree, has shown that the two populations of highand small-amplitude motions respond di erently to hydration of the membrane [25]. These results have quantified the dynamical heterogeneity of the membrane and its dependence on hydration and temperature. The high amplitude motions in the nonharmonic region above the transition at 250 K are not very sensitive to changes in hydration until a high degree is reached where a sharp increase of the <u2> values is seen. In contrast, the small amplitude motions rise continuously with increasing hydration above a threshold
18 Relating Protein Dynamics to Function and Structure |
427 |
of 60% r.h. and above the transition temperature. The hydration dependence of photocycle and pumping characteristics of BR have been correlated with the thermal motions, i.e., below 60% r.h. the BR photocycle can not be completed [32, 33] and proton pumping ceases [27]. It has been shown that not only a global fluidity is necessary for the functioning of BR, but that the particular response to hydration of the di erent populations of motions can be correlated to the functional behavior of BR.
The examination of the lamellar spacing of PM stacks as a function of temperature by neutron di raction has shown that cooling of a PM sample leads to a decrease in the lamellar spacing [34]. The authors suggested that this corresponds to dehydration of PM that takes place at a temperature between 245 and 265 K, depending on the hydrational level of PM. Upon heating, this e ect is reversible and rehydration starts at around 255 K. The initial spacing is reached again at 265–275 K. This process has been put in the context of the dynamical behavior and the transition temperatures of the membranes. In particular, the rehydration has been correlated to the transition temperature around 250 K and the solvent melting e ect observed by R´eat and coworkers [19]. If a hydrated PM stack is flash-cooled, a fraction of the amorphous inter-membrane water turns liquid-like at 200 K during subsequent heating and leaves the intermembrane space without triggering a dynamical transition [35].
18.3.3 Local Core Motions
Local dynamics of the protein core elucidated in more detail the dynamical heterogeneity of BR [19]. For this purpose, a completely deuterated membrane with only the retinal and the amino acids Met and Trp being hydrogenated, has been prepared. These selected groups are located in the retinal binding pocket and the extracellular half of the protein (Fig. 18.3).
The retinal binding pocket is functionally particularly important in the uni-directional proton transfer during the photocycle. The mean square amplitudes <u2> of such a specifically labeled and hydrated PM sample show significantly decreased flexibility compared to the global membrane, with the motions revealed as being more restrained and shielded from solvent melting e ects. Moreover, the investigation of core dynamics in di erent motion regimes (smalland high-amplitude motions) as a function of hydration revealed a particular behavior for the core motions di erent to the one discovered in the membrane globally [36]. This has quantified the dynamical heterogeneity of BR and the results have been interpreted in terms of a more rigid protein core, which meets the functional requirements of the protein.
The retinal binding pocket has to act as a valve between the extracellular and cytoplasmic parts of BR, and, therefore, a certain environmental rigidity is required to control stereo-specific selection of retinal conformations to accomplish the valve function, whilst a certain global fluidity is required which allows large conformational changes during the photocycle.