
Molecular and Cellular Signaling - Martin Beckerman
.pdf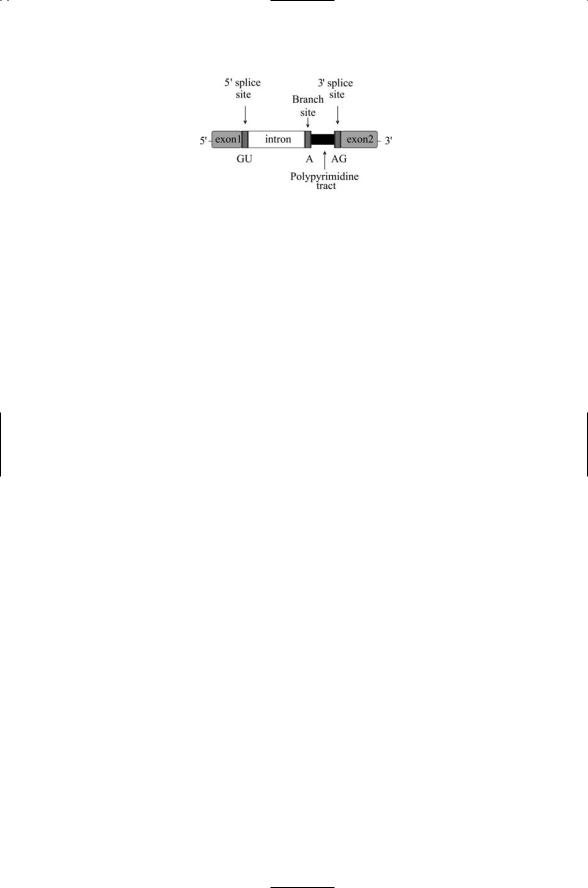
16.13 Small Nuclear RNAs (snRNAs) 401
FIGURE 16.11. Organization of the pre-messenger RNA molecule: A representative pre-messenger RNA molecule is depicted consisting of two exons, an intron, and three accompanying regulatory sites. The introns are usually much longer than the exons, but are shown shortened for illustrative purposes. Nucleotides characteristic of the regulatory sequences are shown at the bottom of the diagram.
The second binding site is the branch site. This site is characterized by the nucleotide sequence YNYURAY, where Y is a pyrimidine. The letter “A” in Figure 16.11 denotes this site. The next portion of the RNA molecule is the pyrimidine tract, which as its name suggests is a string of pyrimidine nucleotides. The last regulatory unit is the 3¢ splice site characterized by either a CAG sequence or a UAG sequence. Splicing is mediated by proteins that bind to particular stretches of DNA just as transcription is mediated by proteins that bind to promoter sites.
16.13 Small Nuclear RNAs (snRNAs)
A family of small nuclear RNA (snRNA) molecules found in the cell nucleus forms the catalytic core of the spliceosome, the name given to the machinery responsible for splicing out introns from the pre-mRNAs. These snRNAs are enriched in uridine and are referred to as the U1 snRNA, U2 snRNA, and so on. The U4 and U6 snRNAs are bound to one another through base pairing and operate as a single unit. The snRNAs function in tight association with from 60 to 100 proteins to form ribonucleoprotein particle (RNP) complexes. These RNP complexes are often referred to as small nuclear ribonucleoprotein particles (snRNPs) or “snurps.” The premRNAs produced during transcription are also complexed with proteins. The combined structures are referred to as heterogeneous nuclear RNP (hnRNP) particles, and the proteins are called hnRNP proteins.
The spliceosome is assembled in several stages. Each stage is identified by the presence of a particular set, or complex, of snRNPs as depicted in
Figure 16.12. The first step in spliceosome assembly is the recruitment of the U1 snRNP to the 5¢ splice site. Several auxiliary proteins assemble at the branch point and polypyrimidine (Py) tract at this time. The first stage where the U1 snRNP binds to the 5¢ splice site sequence is known as the
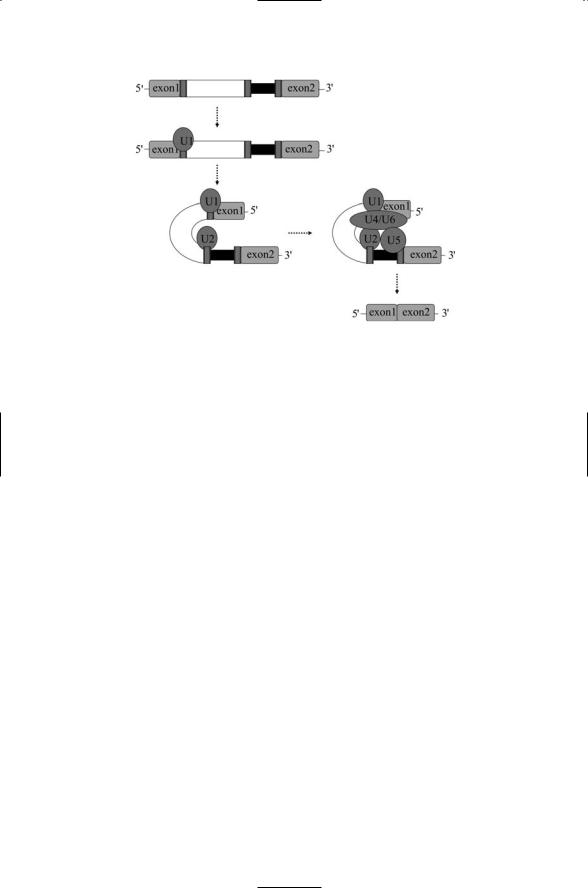
402 16. Gene Regulation in Eukaryotes
FIGURE 16.12. Splicing of a pre-mRNA by the spliceosome: Splicing takes place through the formation of splicing complexes. In the first step, U1 binds to the 5¢ splice site forming the early (E) complex. In the next step, U2 binds the branch point forming the A complex. This action is followed by recruitment of U4/U6 and U5 to form the B complex. U4 and U1 leave and the others complete the splicing process.
commitment stage (complex E). The next stage, the assembly of the U2 snRNP at the branch point, assisted by the auxiliary proteins, is referred to as the presplicing stage (complex A). In the third stage, a preassembled U4/U6 U5 complex is recruited to the U2 snRNP and these components are joined by the U1 snRNP. All components of the spliceosome are now assembled into an early splicing machine (complex B) and, as shown in
Figure 16.12, the 5¢ and 3¢ ends of the intron have been brought together to form a loop.
Once the first phase of the splicing process is complete, the catalytic phase—early splicing and late splicing (complex C)—begins. In the catalytic phase the spliceosome removes the intron and joins the flanking exons. The catalytic process involves several steps and is accompanied by extensive conformational changes and rearrangements of the spliceosome. The U2, U5, and U6 snRNPs form the catalytic core of the spliceosome. These components remain attached to the removed intron, while U1 and U4 leave the spliceosome earlier. At the end of the catalytic stage the introns and Py tracts have been removed leaving the selected set of exons joined together in a mature mRNA molecule.
Transcription and splicing are coupled processes. Splicing begins while the pre-mRNA is still being formed, during the transcription elongation process, rather than after completion. As noted above, long introns separate rather short exons from one another. The coupling of transcription with
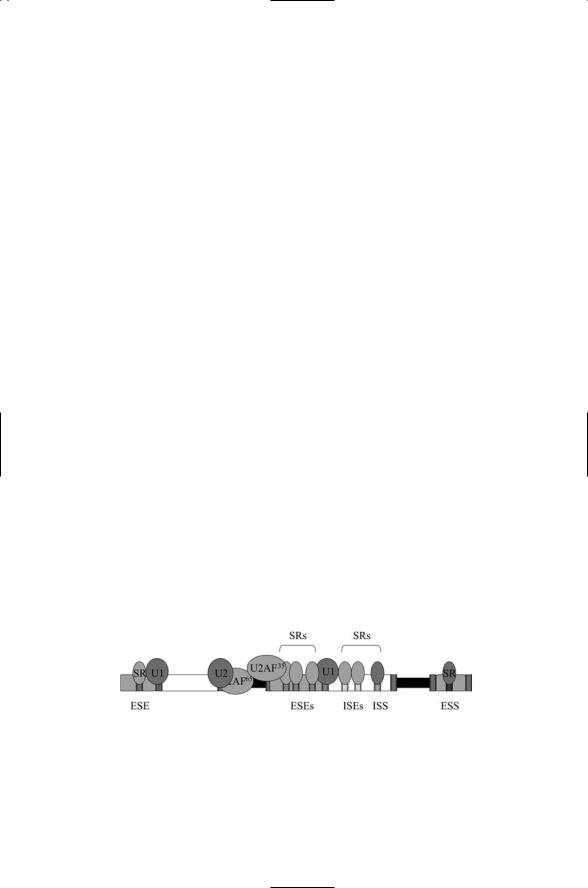
16.14 How Exon Splices Are Determined |
403 |
splicing helps bring together the exons in the correct manner over the large distances and enables components of the transcription machinery to recruit proteins required for splicing.
16.14 How Exon Splices Are Determined
In addition to the principal splice sites shown in Figure 16.11, there are a number of auxiliary splice sites. These auxiliary sites may be located in either exons or in introns and provide places where splicing regulators may bind. Auxiliary sites located within exons are called exonic splice enhancer (ESE) and exonic splice silencer (ESS) sites, depending on which regulatory outcome is supported. Similarly, intronic sites are termed intronic splice enhancer (ISE) and intronic splice silencer (ISS) sites. Two kinds of pro- teins—hnRNPs and SR proteins—bind to these auxiliary regulatory RNA sequences.
SR proteins are a family of essential splicing factors ranging in size from 20 to 75 kDa. Members of the family are characterized by the presence of an arginine-serine-rich (RS) domain of variable length at their carboxyl terminal and one or more RNA recognition motifs (RRMs) in their amino terminal. These proteins are sequence-specific RNA binding proteins that have multiple roles in splicing. They play essential roles in the assembly of the spliceosome at splice sites and in the selection of alternative splice sites.
Figure 16.13 depicts the assembly of a representative set of splicing factors along a section of a pre-mRNA molecule. Two auxiliary splicing factors (U2AF65 and U2AF35) associated with the U2 snRNP have been recruited to the Py tract and 3¢ splice site by SR proteins bound at nearby ESEs. These proteins, and other auxiliary factors, recruit or inhibit components of the spliceosome from assembling at the pre-mRNA and recognizing nearby splice sites. Proteins that bind enhancer or silencer sites can be either SR proteins or hnRNPs.
FIGURE 16.13. SR (serine-arginine-rich) proteins recruit U1 and U2 snRNPs to splice and branch sites: Auxiliary splicing sites and regulatory proteins that bind them determine which exons are spliced together. Abbreviations—Exonic splice enhancer (ESE) site; exonic splice silencer (ESS) site; intronic splice enhancer (ISE) site; intronic splice silencer (ISS) site.
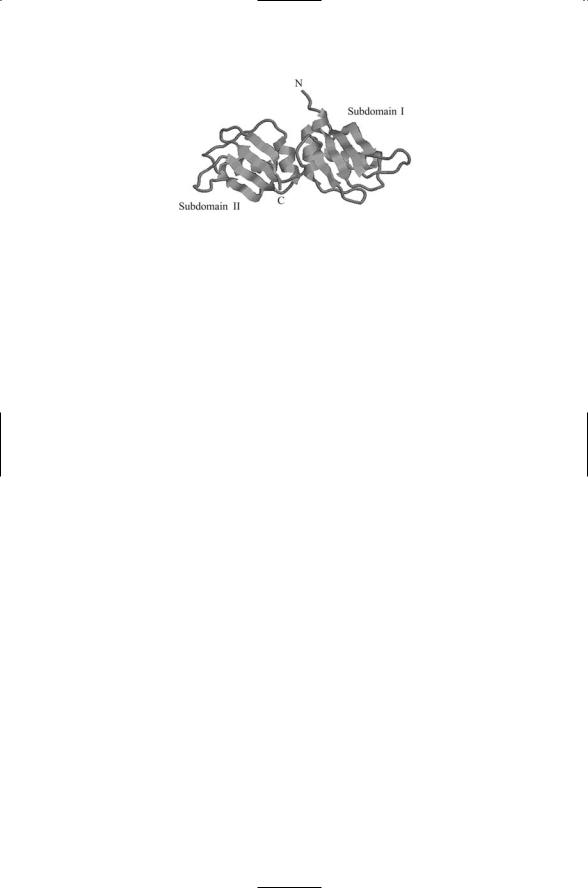
404 16. Gene Regulation in Eukaryotes
FIGURE 16.14. Crystal structure of the N-terminal region (UP1) of the hnRNP A1: Each subdomain contains an RRM and the overall structure of the module is that of an extended RNA binding surface. The crucial amino acid sequences are located on the two middle beta strands of each subdomain. The figure was prepared using Protein Explorer with atomic coordinates deposited in the Brookhaven Protein Data Bank under accession number 1UP1.
Like other regulatory proteins the hnRNP proteins are modular in design. They contain one or more RNA recognition motifs (RRMs) that facilitate their participation in the complexes. An example of an RNA binding domain for an hnRNP called A1 is presented in Figure 16.14. The RNA binding module consists of a pair of subdomains, I and II. Each subdomain consists of a four-stranded antiparallel beta-pleated sheet and two alpha helices off to one side. The overall structure is that of an extended RNA binding surface.
The relative concentration of splicing proteins, and the mix of regulatory sites, jointly determine which combination of exons is assembled into the mature messenger RNA molecule. Different cell types in a multicellular organism will express a different mix of proteins that bind to the auxiliary regulatory sites, and a given cell may alter the mix of splicing regulators over time to produce proteins with slightly altered properties.
16.15Translation Initiation Factors Regulate Start of Translation
The machinery involved in translation initiation serves as an important end point for stress signaling. Before discussing the mechanisms underlying the control of translation it is worthwhile to examine the organization of mRNAs and the machinery that carries out translation. Messenger RNA molecules contain special structures at their 5¢ and 3¢ termini. A cap structure is added to its 5¢ end. The cap consists of a 7-methylguanylate (a CH3 group appended to the 7 position of the G base) plus a triphosphate group that links the 7-methylguanylate to the sugars at the 5¢ end of the RNA molecule. The cap is referred to as an m7GpppN structure, where N is any
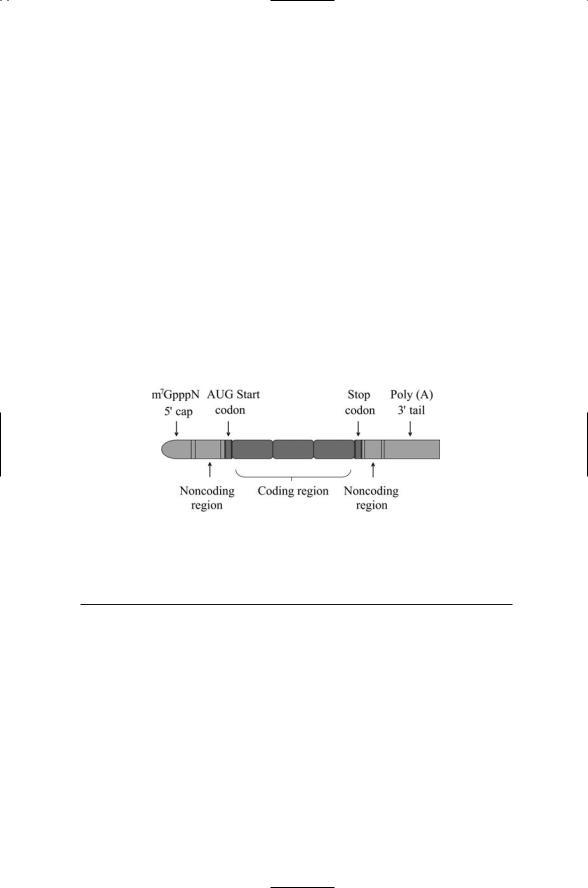
16.15 Translation Initiation Factors Regulate Start of Translation |
405 |
nucleotide. A string of perhaps as many as 200 adenylates is appended to the 3¢ terminus of the mRNA. This addition is referred to as a polyadenylate tail or poly (A) tail. The cap and poly (A) additions are separated from the mRNA coding region by flanking noncoding regions and start and stop codons (Figure 16.15).
A set of proteins called eukaryotic initiation factors, or eIFs, is responsible for assembling the ribosomal 40S and 60S subunits at the mRNA molecule. These proteins are listed in Table 16.5. Translation initiation begins at the 5¢ end of the mRNA. An initiator transfer RNA (tRNA) is a tRNA molecule loaded with a methionine (Met), the amino acid encoded by the AUG start codon. For translation to begin, the Met-tRNA initiator and the small and large ribosomal subunits must be recruited and positioned at the AUG start site of the mRNA. This occurs in a staged manner as shown in Figure 16.16. In the first main step the loaded tRNA molecule and small ribosomal subunit associate. The Met-tRNA molecule is guided to its correct position by the initiation factor eIF2 (eukaryotic initiation factor 2) that forms a complex with the Met-tRNA and a GTP molecule. This terti-
FIGURE 16.15. Organization of the mature mRNA molecule: The coding region is flanked by a pair of noncoding regions, a 5¢ end cap, and a 3¢ end polyadenylate tail.
TABLE 16.5. Vertebrate initiation factors.
Initiator |
Molecular mass |
|
factor |
(kDa) |
Function |
eIF1A |
17 |
Promotes Met-tRNA, 40S, mRNA binding |
eIF2 |
126 |
GTP binding protein, guides Met-tRNA onto 40S |
eIF2B |
277 |
GEF for eIF2 |
eIF3 |
330 |
Binds to 40S, prevents 60S binding |
eIF4F |
291 |
Complex of eIF4E, eIF4G, and eIF4A; eIF4E |
|
|
binds to the cap; eIF4G interacts with eIF3 to |
|
|
augment binding of the preinitiation complex; |
|
|
eIF4A is an RNA-dependent ATPase and an |
|
|
RNA helicase |
eIF4B |
69 |
Facilitates ribosome-mRNA binding |
eIF5 |
45 |
Stimulates hydrolysis of GTP associated with eIF2 |
|
|
|
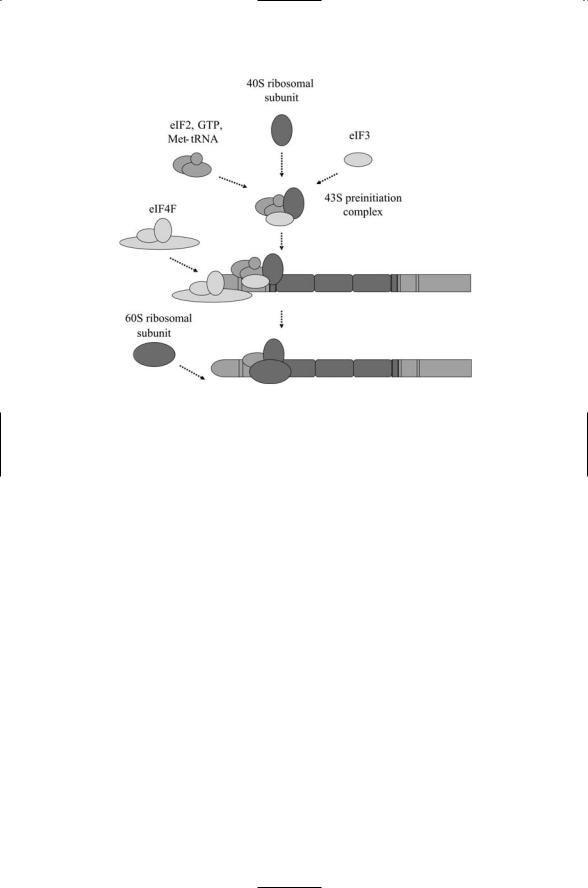
406 16. Gene Regulation in Eukaryotes
FIGURE 16.16. Assembly of the ribosomal 40S and 60S subunits at the mRNA molecule: At the end of the process the 40S subunit, 60S subunit, and Met-tRNA are positioned at the AUG start codon. This positioning is guided by the eIFs with the eIF4E subunit gripping the cap and the eIF4G subunit serving as a scaffold.
ary complex associates with the small (40S) ribosomal subunit to form a 43S preinitiation complex.
Next, the preinitiation complex attaches to the mRNA near its 5¢ end. An initiation factor complex, eIF4F, binds to the m7GpppN cap and prepares the way for the attachment of the preinitiation complex to the mRNA. Upon attachment the preinitiation complex scans in the 5¢ to 3¢ direction for the start AUG codon and halts upon arrival.Another initiation factor,eIF5,stimulates the hydrolysis by eIF2 of GTP to GDP, resulting in the dissociation of the initiating factors from the assemblage leading to the attachment of the large (60S) ribosomal subunit, and translation elongation can begin.
16.16eIF2 Interfaces Upstream Regulatory Signals and the Ribosomal Machinery
The translation initiation factors have a crucial role in guiding the 40S and 60S ribosomal subunits to the start codon. As a result, if the translation initiation factors are prevented from carrying out their tasks, translation will cease. Prevention can be accomplished by phosphorylating certain serine
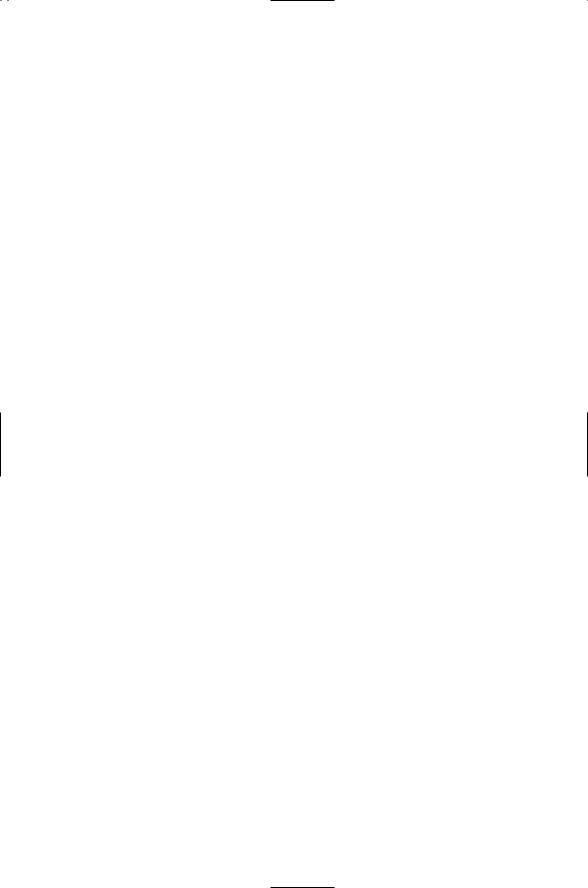
16.17 Critical Control Points for Protein Synthesis |
407 |
residues on eIF2 and eIF2B, thereby placing translation under the firm control of upstream signals conveyed by protein kinases.
The eIF2 translation initiation factor is a GTPase. As noted in Table 16.5, eIF2B functions as a GEF for eIF2, and eIF5 operates as its GAP. The eIF2 initiation factor contains several subunits. Its alpha subunit can be phosphorylated on Ser51 by a number of upstream kinases. One of the kinases that phosphorylates the subunit is protein kinase R (PKR). As discussed earlier in Chapter 9, interferon signaling acts in part through this kinase to halt protein synthesis when a virus infection is detected (through the presence of dsRNAs). Among the other kinases that target eIF2 are GCN2, a kinase that phosphorylates eIF2 in response to poor nutrient conditions, and PERK, a kinase that phosphorylates eIF2 in response to ER stresses.
The eIF2 initiation factor is deactivated by phosphorylation and activated by dephosphorylation. When eIF2 is phosphorylated it binds eIF2B tightly, thereby sequestering both and preventing their translational activities. The translation initiation factor eIF2B catalyzes the conversion of GDP back to GTP, a step that is required for continued cycling and reformation of Met-tRNA-GTP-eIF2 complexes. It, too, can be phosphorylated, by GSK3 among others, to prevent its activities. In response to growth and survival signals, Akt is activated and when this kinase phosphorylates GSK3, eIF2B is freed of the inhibitory activities of GSK3.
16.17 Critical Control Points for Protein Synthesis
The eIF2 and eIF2B proteins are not the only targets of upstream regulatory kinases and phosphatases. The cap-binding, translation initiation factor eIF4E is phosphorylated at Ser209 in response to growth factor, hormonal and mitogenic signaling, and it is dephosphorylated in response to heat shock. Phosphorylation enhances the ability of eIF4E to bind to eIF4G and/or the cap and thus promotes translation. The extent to which eIF4Es are phosphorylated matches the overall translation level at different stages of the cell cycle. These levels are low during G0 phase, increase through G1 and S phases, and then rapidly drop during M phase. Several MAP kinase pathways are involved in phosphorylating eIF4E. These include the Ras- Raf-Mek-Erk that relays growth/mitogenic signals, and the p38 MAP kinase that relays stress signals. Both of these pathways activate Mnk1, a kinase that integrates MAP kinase signals, and in response phosphorylates eIF4E on Ser209.
The cap-binding protein eIF4E is the target of negative regulation by a family of small 10 to 12 kDa-eIF4E binding proteins (4E-BPs).These regulators compete with eIF4G for binding to eIF4E. The ability of the 4E-BPs to compete for binding is dependent on their phosphorylation status. Phosphorylation decreases the binding affinity of the 4E-BPs for eIF4E and leads to dissociation.The best characterized member of the family is 4E-BP1
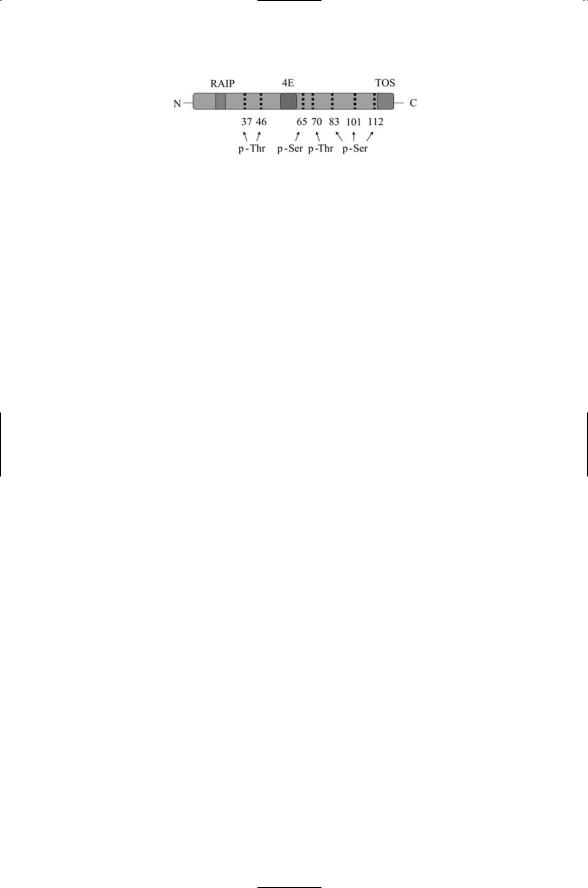
408 16. Gene Regulation in Eukaryotes
FIGURE 16.17. Structure of the human 4E-BP1 protein: The cap-binding protein eIF4E, along with its 4E-BPs, is a critical control point for protein synthesis. The seven identified phosphorylation sites are indicated along with the N-terminal RAIP (Arg-Ala-Ile-Pro) motif, the C-terminal TOS (Phe-Glu-Met-Asp-Ile) motif and the eIF4E binding site. The RAIP and TOS motifs are essential for proper phosphorylation by upstream kinases.
(Figure 16.17). The regulation of eIF4E by 4E-BP1 was discussed in Chapter 6. Recall from that earlier discussion that, under good growth/nutrient conditions, target of rapamycin (TOR) proteins phosphorylate and thus activate a protein called Tap42 that ensures that 4E-BP1 does not bind and inactivate eIF4E (Figure 6.10). Under poor growth conditions Tap42 is not activated by TOR and 4E-BP1 is free to inhibit the activity of the translation initiation factor. The 4E-BP proteins are phosphorylated on a number of serine/threonine residues by several kinases in a hierarchical fashion. For example, they are phosphorylated on Thr37 and Thr46 by Akt activated in the PI3K pathway, and once primed by Akt they are further phosphorylated by hTOR on Ser65,Thr70 and Ser83.These stratified phosphorylation events induce the dissociation of 4E-BP1 from eIF4E.
References and Further Reading
Promoter Architecture
Bell AC, West AG, and Felsenfeld G [2001]. Insulators and boundaries: Versatile regulatory elements in the eukaryotic genome. Science, 291: 447–450.
Blackwood EM, and Kadonaga JT [1998]. Going the distance: A current view of enhancer action. Science, 281: 60–63.
Calhoun VC, Stathopoulos A, and Levine M [2002]. Promoter-proximal tethering elements regulate enhancer-promoter specificity in the Drosophila antennapedia complex. Proc. Natl. Acad. Sci. USA, 99: 9243–9247.
Structure of RNAP II
Cramer P, Bushnell DA, and Kornberg RD [2001]. Structural basis of transcription: RNA polymerase II at 2.8 Ångstrom resolution. Science, 292: 1863–1876.
Gnatt AL, et al. [2001]. Structural basis of transcription: An RNA Polymerase II elongation complex at 3.3 Å resolution. Science, 292: 1876–1882.
Woychik NA, and Hampsey M [2002]. The RNA polymerase II machinery: Structure illuminates function. Cell, 108: 453–463.
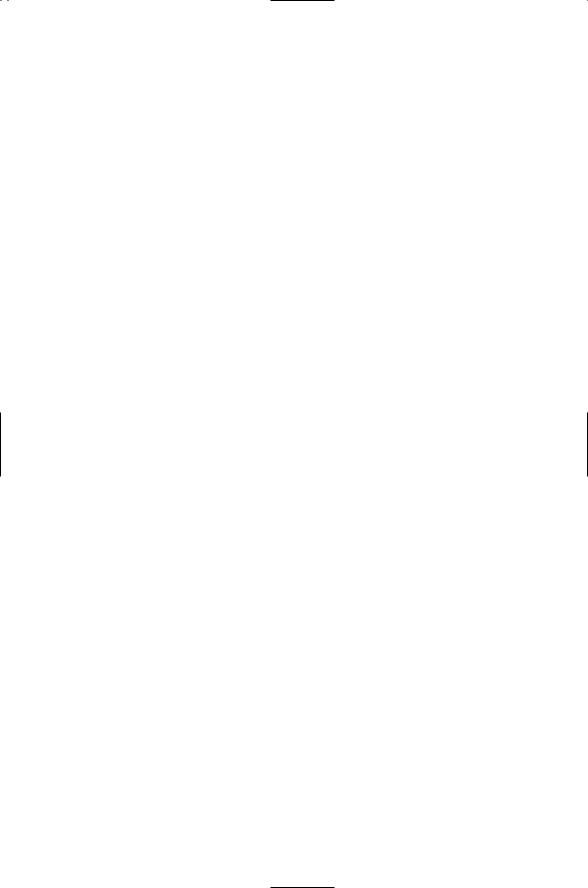
References and Further Reading |
409 |
Chromatin-Modifying Enzymes
Boyer LA, et al. [2000]. Functional delineation of three groups of the ATP-dependent family of chromatin remodeling enzymes. J. Biol. Chem., 275: 18864–18870.
Eissenberg JC [2001]. Molecular biology of the chromo domain: An ancient chromatin module comes of age. Gene, 275: 19–29.
Horn PJ, and Peterson CL [2002]. Chromatin higher order folding: Wrapping up transcription. Science, 297: 1824–1827.
Narlikar GJ, Fan HY, and Kingston RE [2002]. Cooperation between complexes that regulate chromatin structure and transcription. Cell, 108: 475–487.
Vignali M [2000]. ATP-dependent chromatin-remodeling complexes. Mol. Cell Biol., 20: 1899–1910.
Zeng L, and Zhou MM [2002]. Bromodomain: An acetyl-lysine binding domain. FEBS Lett., 513: 124–128.
Mediator Complexes
Asturias FJ, et al. [1999]. Conserved structures of Mediator and RNA polymerase holoenzyme. Science, 283: 985–987.
Boube M, et al. [2002]. Evidence for a mediator of RNA polymerase II transcriptional regulation conserved from yeast to man. Cell, 110: 143–151.
Dotson MR, et al. [2000]. Structural organization of the yeast and mammalian mediator complexes. Proc. Natl. Acad. Sci. USA, 97: 14307–14310.
Lewis BA, and Reinberg D [2003]. The Mediator coactivator complex: Functional and physical roles in transcriptional regulation J. Cell Sci., 116: 3667–3675.
Malik S, and Roeder RG [2000]. Transcriptional regulation through Mediator-like coactivators in yeast and metazoan cells. Trends Biochem. Sci., 25: 277–283.
Taatjes DJ, et al. [2002]. Structure, function, and activator-induced conformations of the CRSP coactivator. Science, 295: 1058–1062.
Theory
Levine M, and Tjian R [2003]. Transcriptional regulation and animal diversity. Nature, 424: 147–151.
Orphanides G, and Reinberg D [2002]. A unified theory of gene expression. Cell, 108: 439–451.
Alternative Splicing
Cáceres JF, and Kornblihtt AR [2002]. Alternative splicing: Multiple control mechanisms and involvement in human disease. Trends Genet., 18: 186–193.
Faustino NA, and Cooper TA [2003]. Pre-mRNA splicing and human disease. Genes Dev., 17: 419–437.
Graveley BR [2000]. Sorting out the complexity of SR protein function. RNA, 6: 1197–1211.
Krecic AM, and Swanson MS [1999]. hnRNP complexes: Composition, structure, and function. Curr. Opin. Cell Biology, 11: 363–371.
Maniatis T, and Reed R [2002]. An extensive network of coupling among gene expression machines. Nature, 416: 499–506.
Smith CWJ, and Valcárcel J [2000]. Alternative pre-mRNA splicing: The logic of combinatorial control. Trends Biochem Sci., 25: 381–388.
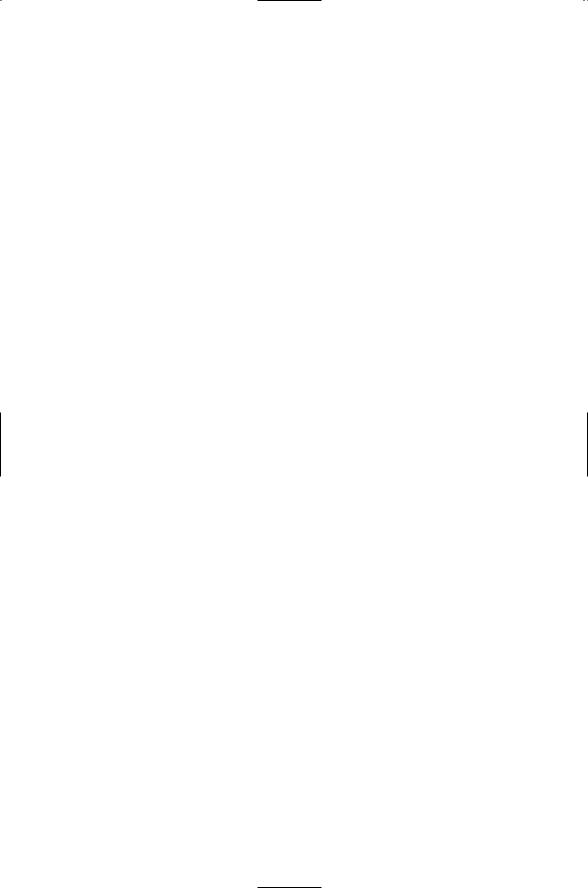
410 16. Gene Regulation in Eukaryotes
Staley JP, and Guthrie C [1998]. Mechanical devices of the spliceosome: Motors, clocks, springs, and things. Cell, 92: 315–326.
Regulation of Translation Initiation
Dever TE [2002]. Gene-specific regulation by general translation factors. Cell, 108: 545–586.
Gingras AC, Raught B, and Sonnenberg N [2001]. Regulation of translation initiation by FRAP/mTOR. Genes Dev., 15: 807–826.
Jacinto E, and Hall MN [2003]. TOR signaling in bugs, brain and brawn. Nature Rev. Mol. Cell Biol., 4: 117–126.
Kosak M [1999]. Initiation of translation in prokaryotes and eukaryotes. Gene, 234: 187–208.
Pain VM [1996]. Initiation of protein synthesis in eukaryotic cells. Eur. J. Biochem., 236: 747–771.
Proud CG [2002]. Regulation of mammalian translation factors by nutrients. Eur. J. Biochem., 269: 5338–5349.
Wang XM, et al. [2003]. The C terminus of initiation factor 4E-binding protein 1 contains multiple regulatory features that influence its function and phosphorylation. Mol. Cell. Biol., 23: 1546–1557.
Problems
16.1Transcription factors and cofactors can promote or inhibit transcription in several ways. List some of these ways. For each way give the location/site of action along the DNA, the specific interaction that mediates the response, and the effect of the binding interaction on transcription.
16.2The translation initiation factor eIF2 is a GTPase. Several different modes of action of GTPases were presented in Chapter 13. For example, Ras acted one way as a switch while Cdc42 cycles continuously, and thus operates in a different manner from Ras. Which of the several GTPase modes of operation does eIF2 follow?
16.3One of the early events in apoptosis is the shutting down of the translation machinery. Give some examples of how the apoptosis regulatory machinery discussed in the last chapter might signal and regulate protein synthesis through interactions of apoptosis signaling/regulatory proteins with the translation initiation factors.