
Molecular and Cellular Signaling - Martin Beckerman
.pdf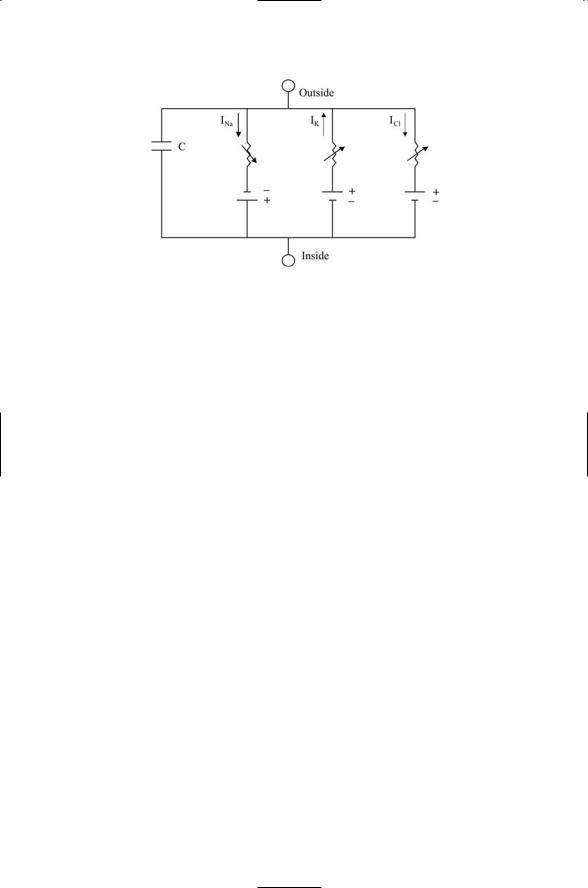
472 19. Ion Channels
FIGURE 19.3. Electrical circuit described by the Hodgkin–Huxley equation: As is customary, the capacitor residing in the capacitive current branch is represented by a pair of short parallel lines, and the batteries in the ionic current branches by pairs of short-long lines denoting cathode (-) and anode (+). Arrows crossing through the resistors symbolize their non-ohmic character. Arrows show the direction of the current flow, sodium and chloride ions inward and potassium ions outward.
with branches for sodium current, potassium current, leakage current, capacitive current, and any external or synaptic currents. If desired, any other ionic currents such as chloride current can be added to the equation in the same way. Ionic currents are small compared to the ionic concentrations outside and inside a neuron and thus do not change the concentrations appreciably. Therefore, the concentration gradients are represented in the circuit diagram by simple batteries that supply currents, one type of battery for each ion species. The voltage across the terminals in these batteries is the reversal potential for that ion species represented by the Nernst equation: approximately +60 mV for sodium, -90 mV for potassium, and
-75 mV for chloride. Time and voltage-varying resistors are present in each branch of the circuit.
19.4 Ion Channels Have Gates that Open and Close
The non-ohmic character of membrane resistance is of considerable significance. In a non-ohmic, or rectifying, resistor, the flow of ions through a particular kind of ion channel is directional. That is, ions will pass through the ion channels in one direction more easily than in the other direction. If the ions pass more easily into the cell, the ion channel is inward rectifying, and if they pass more readily out of the cell, the ion channel is outward rectifying. The rectifying character of the membrane permeability naturally leads to the introduction of gates that open and close to permit the flow of ions through the channels.
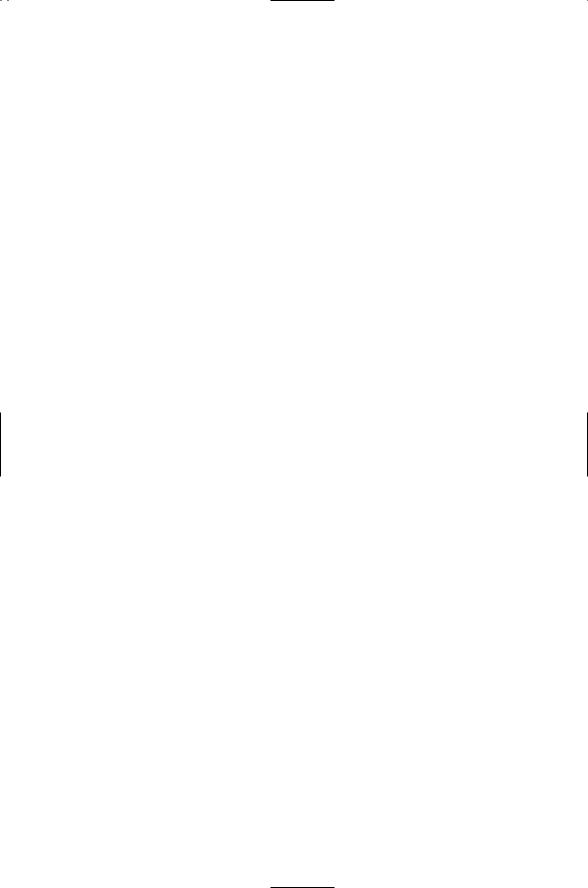
19.4 Ion Channels Have Gates that Open and Close |
473 |
To model the opening and closing of sodium and potassium channels in response to depolarization of the nerve membrane, Hodgkin and Huxley introduced three gates. Recall that the sodium channels rapidly open as the membrane is depolarized and then shut after a short time interval. Hodgkin and Huxley describe this behavior in terms of two gates: an activation gate and an inactivation gate. Initially, at the resting membrane potential, the activation gate is shut and the inactivation gate is open. Once the threshold depolarization, for activation is exceeded, the activation gate opens allowing sodium ions to enter the cell. Further increases in depolarization allow the activation gates to open fully, but the inactivation gate, which operates more slowly, closes in response to the increasing depolarization, thereby shutting down the flow of sodium ions.
The third gate introduced by Hodgkin and Huxley is the activation gate for potassium. This gate is also opened by depolarization, but much more slowly than the activation gate for sodium. As the gate for the potassium channel opens in response to depolarization, potassium ions leave the cell, thus opposing the effect of the sodium entry, and eventually overcoming it to repolarize the cell. The repolarization accelerates the further closing of sodium channels and leads to the hyperpolarization of the membrane. When this occurs the inactivation gate is opened; the activation gate is shut, and the membrane relaxes back to the resting potential, ready for another round of excitation.
Activation and inactivation gates have sigmoidal (S-shaped) dependencies on voltage. The differences between activation and inactivation gates are illustrated in Figure 19.4. As shown in the figure, inactivation gates open when the membrane is hyperpolarized and shut when the membrane is depolarized. Activation gates work the opposite way. They open when the membrane is depolarized and shut when the membrane is hyperpolarized. Hodgkin and Huxley called the sodium and potassium gates m-, h-, and n-gates. That is, a fast m-gate describes the activation process and a slow h-gate describes the inactivation process for the sodium entry, while the potassium channels are controlled by a single n-gate. The sigmoidal dependence of their opening and closing was approximated by algebraic terms— cubic for the m-gate, linear for the h-gate, and quadratic for the n-gate. In mathematical terms, dimensionless variables m, h, and n were introduced, each a function of Vm. These variables assume values between 0 and 1 corresponding to completely closed and completely open. The sodium conductance was expressed as
gNa = |
|
Na m3 h. |
(19.8) |
||
g |
|||||
Similarly, the potassium conductance was written as |
|
||||
gK = |
|
|
K n4 . |
(19.9) |
|
g |
The quantities m3h and n4 represent the fractions of open sodium and potassium channels, respectively, and the g-bars are constants representing the
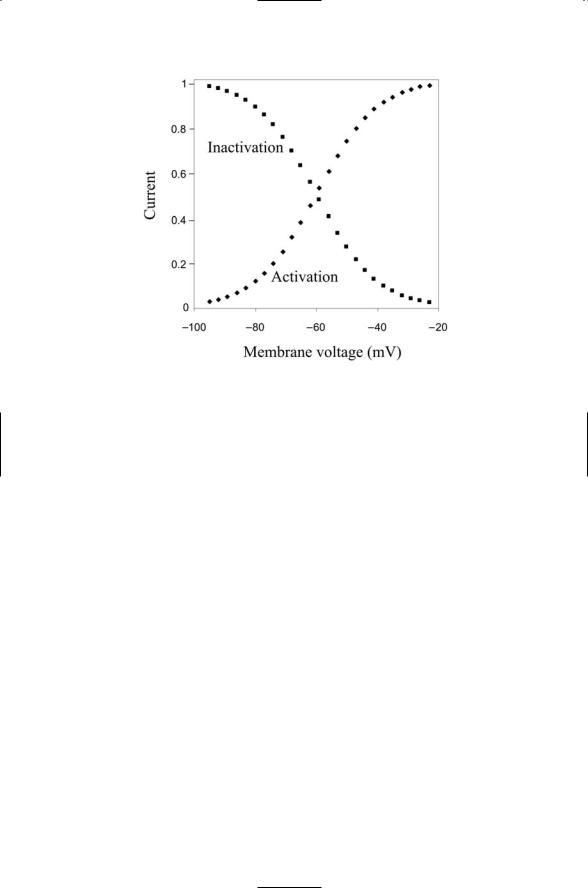
474 19. Ion Channels
FIGURE 19.4. Activation and inactivation functions and resulting membrane currents: The activation (diamonds) and inactivation (squares) functions represent the amount of current flowing through the ion channels relative to the maximum possible currents. These quantities are plotted as functions of the membrane voltage.
maximum conductances occurring when the channels are completely open. These core equations are supplemented by a set of rate constants and rate equations for the m-, h-, and n-gate variables.
19.5 Families of Ion Channels Expressed in Plasma Membrane of Neurons
The human genome contains hundreds of genes that encode ion channel subunits. The repertoire of ion channels is further increased through extensive use of alternative splicing. Still more ion channel forms are generated by mixing together different combinations of subunits. The resulting ion channels differ from one another in their biophysical properties in several ways. The differ in their
•ion selectivity and direction of ion movement,
•gating mechanism,
•topology and assembly plan,
•time course of activation and inactivation, and
•reversal potentials for activation and inactivation.
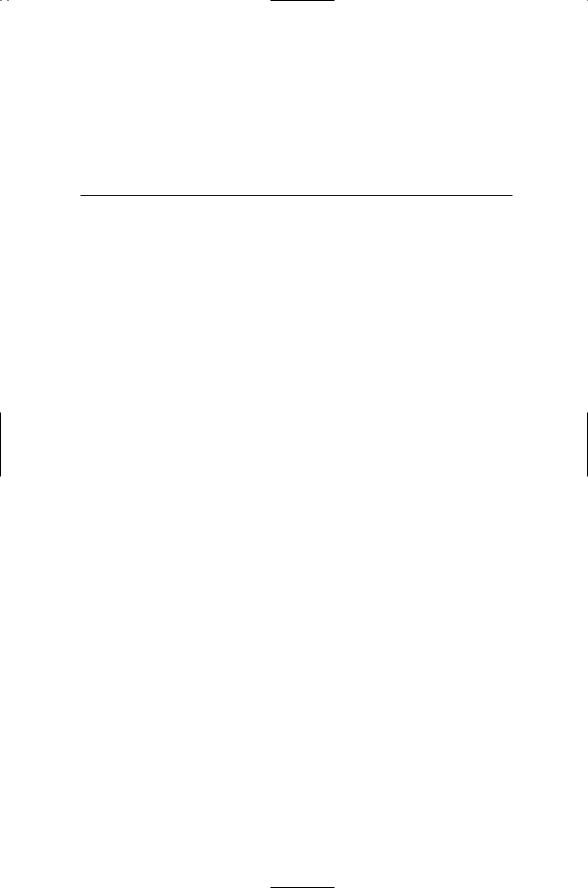
19.5 Families of Ion Channels Expressed in Plasma Membrane |
475 |
TABLE 19.2. The four families of ion channels found in the plasma membrane of neurons: Except for the outward directed potassium channels, ion channels allow for the inward selective diffusion of the ion species listed in the second column. Abbreviations—Hyperpolarization-activated cyclic nucleotide gated (HCN); chloride channel of the CLC family (ClC); nicotinic acetylcholine receptor (nAChR); g-aminobutyric acid Type A, Type C (GABAA,C); 5-hydroxytryptamine Type 3 (5-HT3); a-amino-3-hydroxyl-5-methyl-4-isoxazole propionate acid (AMPA); N-methyl-D-aspartate (NMDA).
Ion channel |
Selectivity |
Subunit topology |
Channel assembly |
6TM cation |
|
6TM, loop |
Tetrameric |
|
Ca2+ |
24TM, 4 loops |
Monomeric |
Calcium |
|
|
|
HCN |
Na+, K+ |
|
|
Potassium |
K+ |
|
|
Sodium |
Na+ |
|
|
Voltage-gated anion |
Cl- |
18IM |
Dimeric |
ClC |
|
|
|
Cys-loop receptor |
|
4TM |
Pentameric |
nAChR |
Cations |
|
|
GABAA,C |
Anions (Cl-) |
|
|
Glycine |
Anions (Cl-) |
|
|
5-HT3 |
Cations |
|
|
Glutamate receptor |
Na+, K+ |
3TM, loop |
Tetrameric |
AMPA |
|
|
|
kainate |
Na+, K+ |
|
|
NMDA |
Ca2+ |
|
|
|
|
|
|
Ion channels have several central properties. One of these is selectivity— the ability to pass some ion species through the membrane rapidly while preventing passage of other ion species. A closely related property is direction of ion movement. Ion channels let ions pass through in one way only.
Some let ions into the cell while others let ions leave the cell, but none allow two-way passage. Another essential property of an ion channel is gating— the ability to open and close the pore through which the selected ions pass in response to voltage across the membrane and to ligands, intracellular and extracellular.
The different kinds of ion channels can be grouped into four large families. As can be seen in Table 19.2, two of the families open and close in response to changes in membrane voltage, and are thus said to voltagegated. One of these families permits the selective passage of cations and the other family allows the passage of anions. The other two families are gated by ligands. For that reason they are designated as receptor ion channels. The voltage-gated ion channels, of which there are many different kinds, can be further distinguished by their time courses for activation and deactivation, and by their reversal potential. That is, by how fast they open and close, and at which membrane voltages, as was discussed earlier in the chapter. Finally,
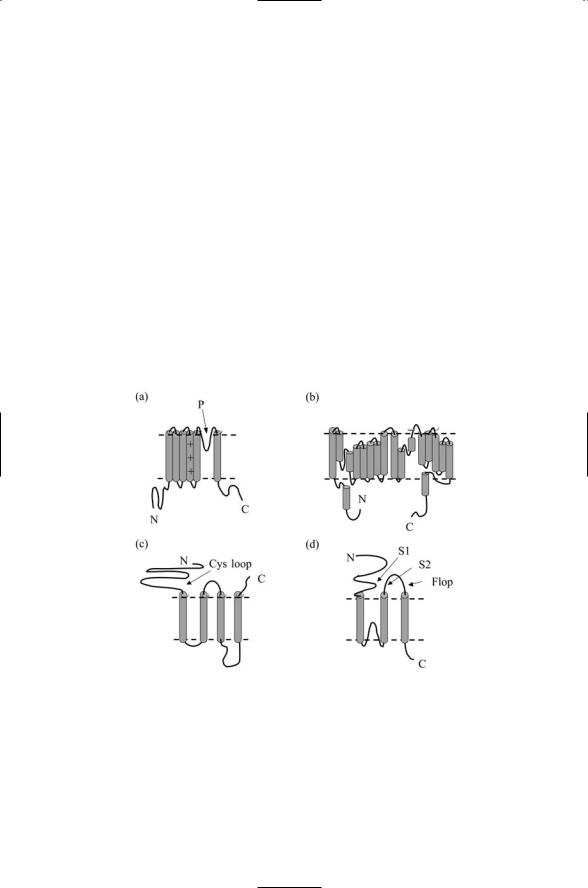
476 19. Ion Channels
the different families of ion channels are characterized by their subunit composition (assembly plan) and by the manner in which the polypeptide chain for each subunit threads back and forth through the membrane (topology).
19.6 Assembly of Ion Channels
Ion channels are assembled from multiple subunits arranged in regular ways to form pores through which the ions diffuse. The threading patterns characteristic of the subunits belonging to each of the four families of ion channels are presented in Figure 19.5. In the case of the voltage-gated cation channels, the core unit, or basic module, consists of a polypeptide segment that passes back and forth through the plasma membrane six times so that the N- and C-terminals are both on the intracellular (cytoplasmic) side. As illustrated in Figure 19.5(a), there is a loop (helix) between the fifth the sixth transmembrane segments (TM5, TM6). The loop regions from each subunit jointly form the conducting pore and selectivity filter (the nar-
FIGURE 19.5. Ion channel subunit threading patterns: (a) Voltage-gated potassium channel—Transmembrane helices 5 and 6 along with the indicated loop form a conducting pore. The positively charged residues in transmembrane segment 4 plus portions of transmembrane helices 1–3 form the voltage sensor. (b) Bacterial ClC showing the threading of 18 alpha helices of varying lengths. (c) Acetylcholine receptor ion channel indicating the presence in the amino terminal portion of a conserved Cys loop near the membrane surface. (d) AMPA receptor ion channel showing the locations of S1 and S2 segments, which, along with the Flop segment, form the ligand-binding domain.
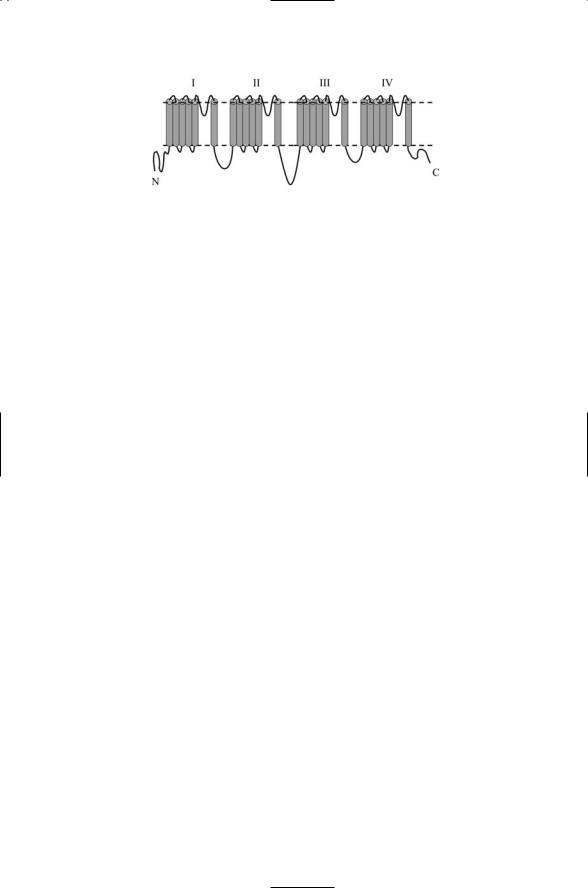
19.6 Assembly of Ion Channels |
477 |
FIGURE 19.6. Single chain voltage-gated cation channel topology: Shown in the figure is a single 6 ¥ 4-pass polypeptide chain topology characteristic of bacterial KscA potassium channel.
rowest part of the pore) that allows certain ion species to flow through. In channels that are voltage-gated, transmembrane segment 4 (TM4) contains a number of positive changes (basic arginine or lysine amino acid residues), and along with elements of TM1–3 functions as the voltage sensor. In channels whose gates are controlled by intracellular ligands, binding sites for the regulators are location on the C-terminal segment that follows TM6.
Voltage-gated cation channels are assembled in one of two ways. In the Shaker family of voltage-gated potassium channels, four of six TM core units are arranged into the ring. In many voltage-gated sodium channels and voltage-gated calcium channels, the amino acid residues replicated among the four subunits form domains belonging to a single, long (2000 amino acid residues), 6 ¥ 4 = 24-pass, polypeptide chain. In either assembly mode, the result is a fourfold symmetric ring structure in the plasma membrane with a pore in the center. A typical threading pattern for a 24-pass transmembrane protein, the bacterial KscA potassium channel, is presented in Figure 19.6.
The threading pattern and assembly plan for voltage-gated anion channels are radically different from those of the cation channels. As shown in
Figure 19.5(b), the polypeptide chain of a ClC chloride channel subunit consists of 18 distinct alpha helices of varying lengths. Some of the helices pass through the membrane, but others do not and one helix resides entirely in the cytoplasm. As will be discussed shortly, the anion channel is formed by two of these subunits, and in place of a single pore in the center there are two off-center pores.
The two families of ligand-gated ion channels can be further divided into several smaller families according to the types of ligands, or neurotransmitters, being bound. Some ligand-gated ion channels permit the passage into the cell of cations, while others allow anions such as chloride to enter the cell. When an anion enters the cell the net charge inside the cell becomes more negative and the membrane voltage shifts to more hyperpolarized values. Since action potentials are generated by threshold-exceeding depolarizations, the entry of negative ions such as chlorine suppresses firing. Neurotransmitters such as GABAA,C and glycine that act through anionic receptors are referred to as inhibitory neurotransmitters. Conversely,
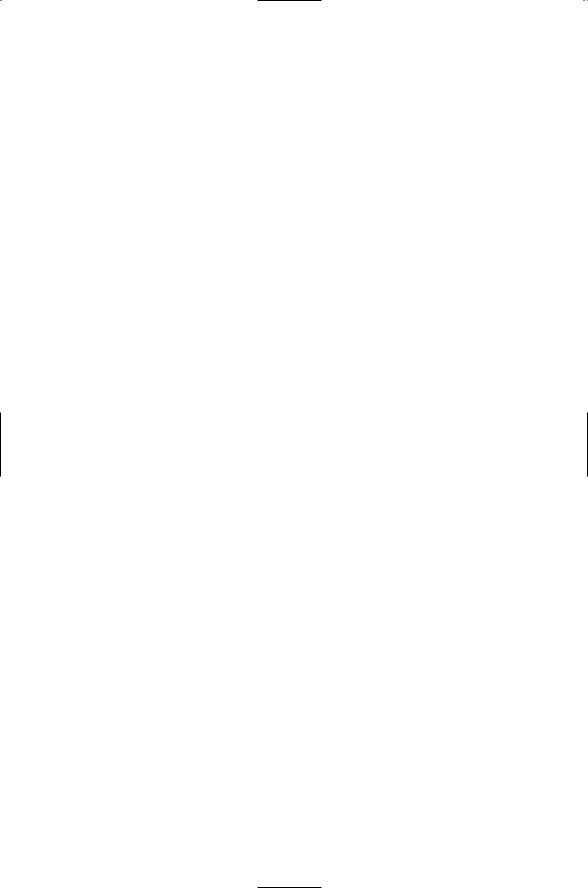
478 19. Ion Channels
neurotransmitters such as glutamate and acetylcholine that act though cationic receptors are referred to as excitatory neurotranmitters.
19.7 Design and Function of Ion Channels
Ion channels are modular in design and function as small molecular machines. Voltage-gated ion channels are modular in design. Each ion channel is a small molecular machine with core (alpha) subunits that form its pore, voltage sensor, and selectivity filter. Voltage-gated sodium, potassium, and calcium channels possess one or two regulatory b subunits. Calcium channels contain not only a 6TM a1 core subunit and a cytoplasmic b subunit, but also an extracellular a2 subunit linked to a single-pass transmembrane d subunit by disulfide bridges (the a2-d subunit), and, in skeletal muscle and some neurons, a 4TM g subunit. These subunits all have regulatory roles in the operation of the channels.
The gates of the ion channels are electroand chemo-mechanical devices that open and close by means of conformational changes. The voltage sensor (or ligand-binding domain) is connected to the helices forming the pore. In response to voltage changes (or ligand binding), the sensor module does mechanical work on the helices lining the pore to alter its conformation from a closed to open one. A variety of regulatory mechanisms are encountered in the various ion channels. These include direction-sensitive blocking elements and regulation by second messengers, G protein bg subunits, and protein kinases and phosphatases.
Receptor ion channels alter conformation in response to ligand binding. As was the case for other signaling receptors, the underlying mechanism is usually an allosteric one. The receptors contain multiple ligand-binding sites. Binding of a ligand at a regulatory site in the protein changes the electrostatic environment resulting in the stabilization of the protein in a different subset of conformations. As a result the binding properties at active sites are changed in a reversible way. The mechanism is an indirect one in that binding at the regulatory site influences binding at a physically removed primary, or active, site through conformational changes rather than by direct physical contact.
19.8 Gates and Filters in Potassium Channels
Potassium channels have spatially separated voltage gates and selectivity
filters. A crucial feature of a voltage sensitive ion channel is its ability to sense changes in voltage and use these changes in voltage as an energy source to drive the opening and closing of the channel gate. The threedimensional atomic structures of several potassium channels have been determined in a series of landmark studies. The resulting data reveal how
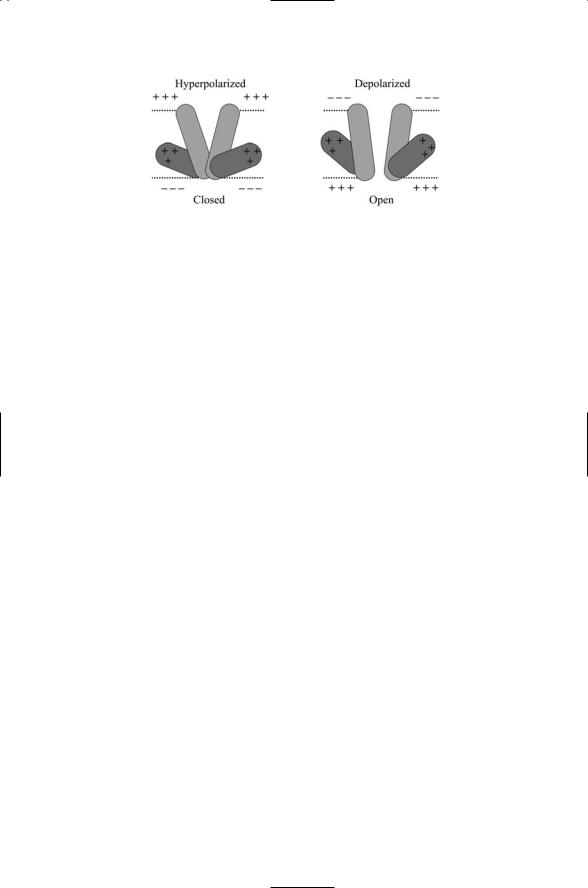
19.9 Voltage-Gated Chloride Channels Form a Double-Barreled Pore |
479 |
FIGURE 19.7. Potassium channel voltage sensor: In the absence of depolarizing currents, the membrane is hyperpolarized. The positive charges in helix S4, along with the other components of the S1 to S4 voltage sensor, maintain the channel in a closed configuration. When depolarizing currents are present the S1 to S4 helices move towards the outer leaflet, and as a result the pore helices S5 and S6 shift their orientation, thereby opening the pore.
the channels open and close in response to changes in membrane voltage and how these machines selectively permit potassium ions to flow through.
The representation of the subunit as being composed of equal-sized helices each passing through the membrane is a simplification that emphasizes the sequential connections between the helices. In the threedimensional structure of the subunit some of the helices are not only not perpendicular to the plane of the membrane but actually lie within the membrane parallel to its surfaces. The voltage-operate gate works in the following way. Charged groups in S4 together with a portion of S3 and the loop connecting it to S4 form a charged paddle. This paddle lies entirely within the lipid bilayer, and changes its orientation in response to changes in membrane voltage. As illustrated in Figure 19.7, when the membrane is depolarized the paddle tugs on other helices pulling open the pore to let the ions through.
The selectivity filter is separated in position from the gate. The subunit 6-pass chain contains an intramembrane loop between segments 5 and 6. These last two segments and the loop from each of the four subunits of the protein form the ion selectivity filter and pore. As can be seen in Figure 19.8, the overall shape of the channel is that of an inverted tepee with the broad portion including the intramembrane loops near the top and the narrowest portion at the bottom.
19.9 Voltage-Gated Chloride Channels Form a
Double-Barreled Pore
The second set of voltage-gated ion channels is the anion, or chloride, channel family. These ion channels are often referred to as the ClC family to distinguish them from the cystic fibrosis transmembrane conductance
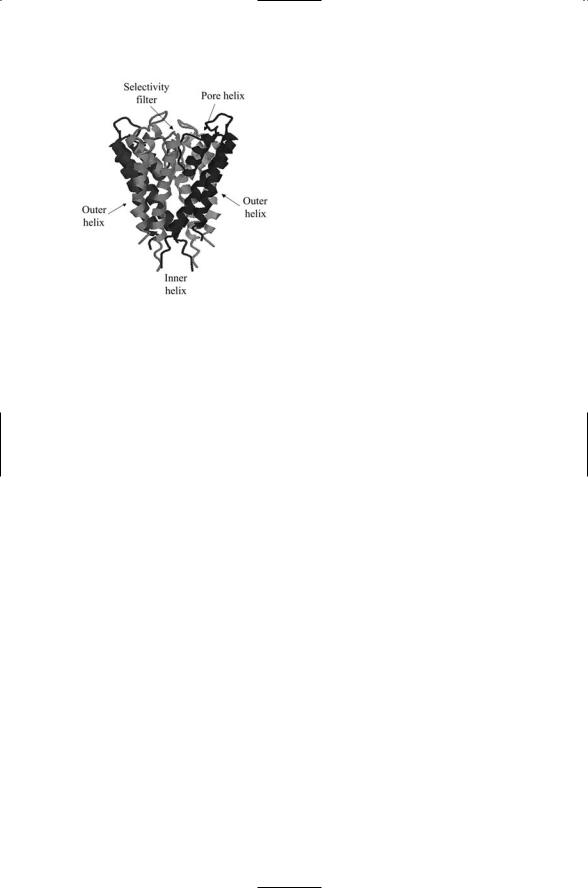
480 19. Ion Channels
FIGURE 19.8. Potassium channel pore: Shown is the tetramer formed by membranespanning S5 and S6 segments and connecting intracellular loop from each domain from a bacterial (KscA) potassium channel. The data determined by means of X-ray crystallography were rendered as a ribbon diagram showing the a-helices and connecting loops. The top of the tetramer lies in the extracellular space and the bottom is in the intracellular region. The figure was generated using Protein Explorer with atomic coordinates deposited in the Brookhaven Protein Data Bank under accession code 1BL8.
regulator (CFTR) chloride channels implicated in cystic fibrosis, and the GABA and glycine receptors, which are ligand-gated and not voltage-gated. The ClC ion channels are found in the plasma membrane and in the membranes of internal organelles. These anion channels are not responsible for generating action potentials, but instead regulate the excitability of muscle and nerve cells, the acidification of internal organelles, and cell volume.
The X-ray crystal structure of two bacterial ClC family members reveal a radically different architecture and pore structure from the voltage-gated cation channels. In place of the four (or five) subunits used to form a centrally positioned conducting pore, only two subunits are utilized. Each subunit is quite large, and consists of 18 a-helices, some short and others long enough to pass through the membrane. Each subunit is large enough to form its own pore, and two of these subunits assemble into the chloride ion channel.
A side view of the ion channel formed by a pair of identical subunits is presented in Figure 19.9. As can be seen, the channel possesses a pair of off-center pores. As was the case for the cation channels the helices are oriented in a variety of ways. These varied orientations are not arbitrary but instead bring together the charged residues in the right way, from different positions in the polypeptide chain, to form the gate and selectivity filter.
19.10 Nicotinic Acetylcholine Receptors Are
Ligand-Gated Ion Channels
Ligand binding controls the gates in the other two superfamilies of ion channels. One of these families is the cys-loop, or acetylcholine, family. In this family, the polypeptide chains comprising the subunits pass through the plasma membrane four times. The name for this family is derived from one
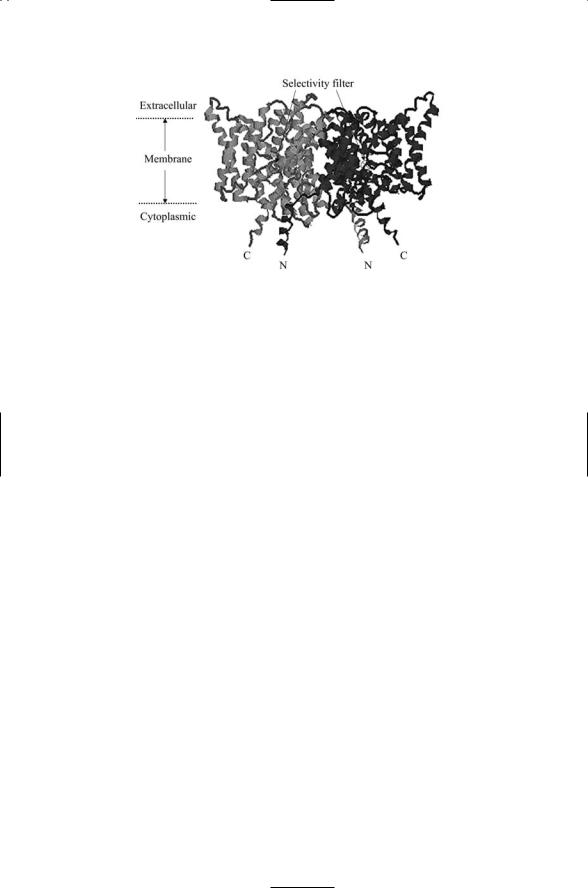
19.10 Nicotinic Acetylcholine Receptors Are Ligand-Gated Ion Channels |
481 |
FIGURE 19.9. The ClC chloride ion channel determined by X-ray crystallography: A side view of the dimeric chloride-selective ion channel is presented. The approximate locations of the selectivity filter in the two subunits are denoted by the circled Xs. The figure was generated using Protein Explorer with atomic coordinates deposited in the Brookhaven Protein Data Bank under accession code 1KPK.
of the structural properties of the subunits. Their extracellular amino terminal contains a cys-loop—a pair of disulfide-bridged cysteines separated by a stretch of 13 amino acid residues.
Nicotinic acetylcholine receptor ion channels are responsible for excitatory signaling at the connections between nerve and muscle cells, or neuromusclular junctions, located throughout the body. There are two types of acetylcholine receptor ion channels—nicotinic and muscarinic. The former bind to the nicotine whereas the latter binds to the muscarine, like nicotine an alkaloid. Those nicotinic receptors, located at neuromuscular junctions, are termed muscle-type nAChRs, while those found at neuron-to-neuron contacts in the spinal cord ganglia and in the brain are referred to as neuronal nAChRs. As their name indicates, the neuronal nicotinic receptors mediate nicotine addiction in smokers, whereas muscle-relaxing, paralyzing, and spasm-promoting toxins target neuromuscular nAChRs. Examples of toxins that target nicotinic acetylcholine receptors are atropine, curare, and scopolamine, all from plants, and cone snail and cobra toxins.
The nAChRs are pentamers, assembled in a symmetric or nearly symmetric ring with a pore in the center. There are 17 known vertebrate subunits. These subunits belong to one of five groups—a, b, g, d, and e. Neuronal receptors are typically homopentamers of a particular a subunit or heteropentamers of a and b subunits. The muscle-type of nAChR is less varied.
These receptors consist of two a1 subunits, plus one each of a b1, d, and g or e subunit.
Perhaps more has been learned about the acetylcholine receptor than any other neurotransmitter-gated ion channel. It has been studied with