
Molecular and Cellular Signaling - Martin Beckerman
.pdf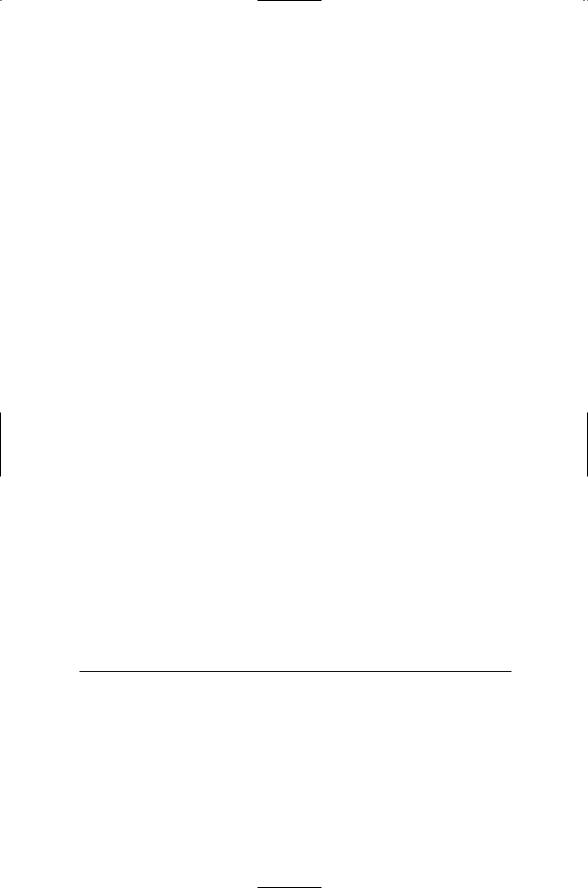
502 20. Neural Rhythms
determined by the synaptic connections and by the mix of ion channels expressed by each neuron.
Two kinds of neurotransmitters are released at the presynaptic termi- nals—glutamate and acetylcholine. These neurotransmitters diffuse across the synaptic cleft and bind to receptors expressed on the postsynaptic cell. In crustaceans, glutamate and acetylcholine both act as inhibitory, not excitatory, neurotransmitters. The glutamate receptors are members of an inhibitory glutamate receptor ion channel (IGluR) family. They resemble the GABA and glycine receptors, and open to permit the entry of Cl- ions into the cell, as do the crustacean acetylcholine receptor ion channels.
20.11 CPGs Have a Number of Common Features
Many of the key design elements found in the stomatogastric ganglion (STG) pyloric circuit are encountered in other central pattern generators. Some of these properties, such as the occurrence of certain sets of ion channels, operate at the cellular level. Other properties, such as mutual inhibition, are related to how the circuits are built, and operate at the network level. A summary of design properties common to many CPGs is presented in Table 20.3.
One of the most common properties is spike frequency adaptation. Small conductance, calcium activated potassium channels are quite sensitive to small changes in calcium concentration. Although the effect of sodium and potassium is small compared to their concentrations within the cell, the same is not true for calcium. Its concentration in the cell in quite low, and small changes in calcium concentration can be sensed and responded to. The small conductance, calcium-activated potassium channels open in response to changes in calcium concentration. The effect of these openings is to hyperpolarize the membrane and decrease its excitability in response to sustained firing. The sequence of pulses exhibits an increasing time lag between successive pulses, and eventually reaches a steady state firing frequency.
TABLE 20.3. Amine and peptide neuromodulators expressed in humans: Abbreviations for specific neuromodulators (excepting the general opioid and tachykinin categories) are shown in parentheses.
Amines |
Peptides |
Acetylcholine (ACh) |
Corticotropin-releasing factor (CRF) |
Dopamine (DA) |
Cortistatin (CST) |
Epinephrine (E) |
Opioids (dynorphins, endorphins) |
Histamine (HA) |
Oxytocin (OT) |
Norepinephrine (NE) |
Somatostatin (SST) |
Octopamine (OCT) |
Tachykinins (substance P, neurokinins) |
Serotonin (5-HT) |
Vasopressin (AVP) |
|
|
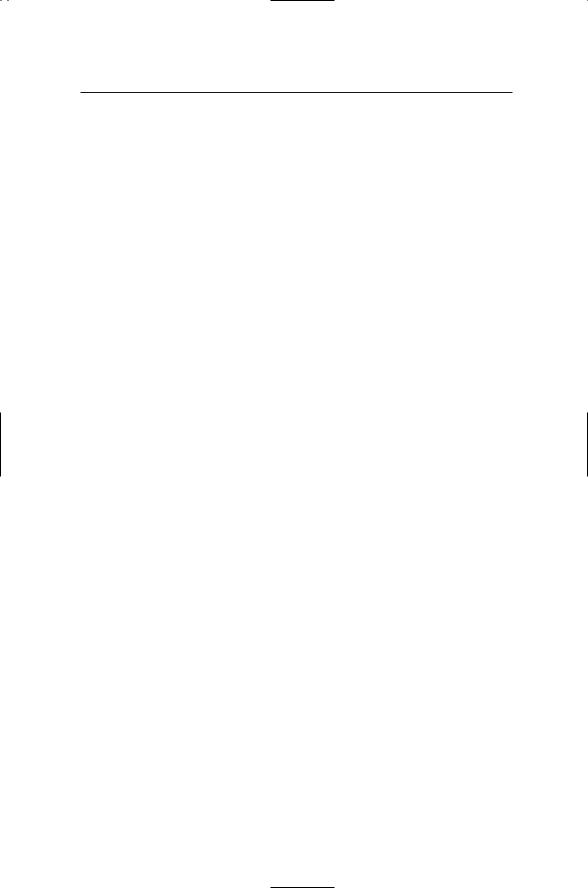
20.11 CPGs Have a Number of Common Features 503
TABLE 20.4. Properties of central pattern generators.
Property |
Description |
Cellular |
|
Rhythmic bursting |
Pacemaker and other rhythm-producing |
|
neurons express HCN ion channels that |
|
support rhythmic bursting |
Plateau potentials |
Increase excitability resulting from a stable |
|
state lying close to thresholds for action |
|
potential generation |
Spike frequency adaptation |
Small conductance, calcium-activated |
|
potassium channels allow for adaptation of |
|
the firing rate |
Post-inhibitory rebound |
Low voltage-activated (T-type) calcium |
|
channels permit the temporary increase in |
|
excitability |
Network |
|
Input signals that initiate rhythms |
Input from sensory neurons, and from neurons |
|
in brain stem control areas |
Feedback signals that regulate |
Mechanical and chemical signals that are used |
rhythms |
to fine tune the rhythms |
Highly interconnected circuits that |
Mutual inhibition and gap junctions ensure the |
generate rhythms |
proper firing sequences and produce synchrony |
Pacemaker cells that generate |
Cells that entrain other cells in the CPG |
rhythms |
|
Regulatory |
|
Extrinsic |
Neuromodulatory signals sent from neurons |
|
outside the pattern generation circuit |
Intrinsic |
Neuromodulatory signals sent and received |
|
within the rhythm-producing circuit |
|
|
Reciprocal (mutual) inhibition is another widely encountered network property. The underlying phenomenon is a simple one. Suppose that two cells, A and B, expressing the ion channels mentioned in Table 20.4, mutually inhibit one another. Further suppose that A fires first. It will inhibit B from firing while it is bursting, but then will exhibit spike frequency adaptation or simply cease to fire. This cessation will release B from its inhibition. B will fire and inhibit A from firing until it too has completed its firing sequence. A will then be released from inhibition and the cycle will repeat. Alternatively, if the B cell being inhibited exhibits a gradual depolarization of its membrane, as illustrated in Figure 20.8, it will escape from the inhibition when its membrane potential depolarizes sufficiently. When it starts firing it will inhibit the A cell from firing and shut down its burst. This will continue until the membrane of the A cell depolarizes and so on.
Another property commonly encountered in rhythmically firing neurons is the presence of plateau potentials. Many motor neurons exhibit bistability. Recall that stable states are long-lived states. If a system is in a stable state it will return to that state whenever it is subjected to small perturba-
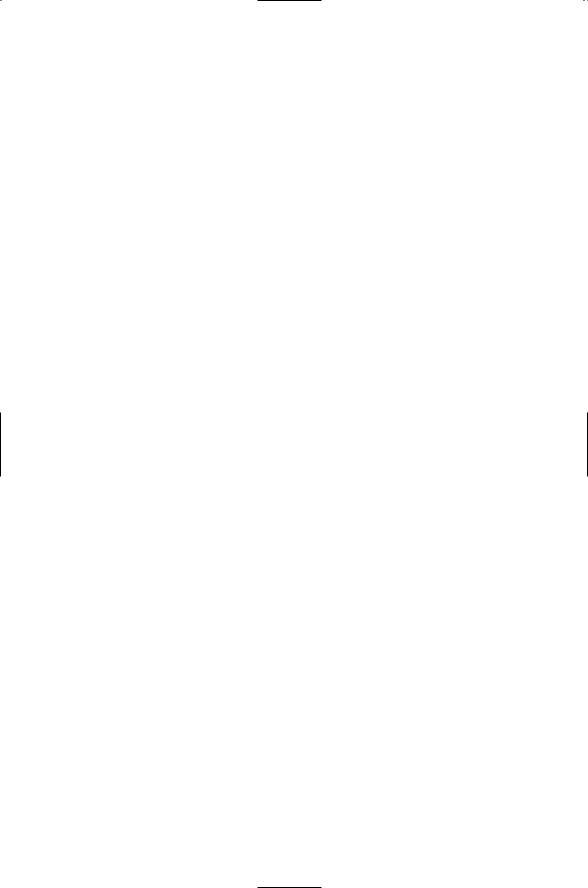
504 20. Neural Rhythms
tions and disturbances. Plateau potentials are membrane potentials that, like the resting membrane potentials, are stable states. Plateau states occur at depolarizations greater than that of the resting membrane potential. When a membrane is at a plateau potential, it is far more able to repeatedly fire action potentials, even in the absence of sustained excitatory input from synapses. As a result neurons possessing plateau potentials exhibit two types of behavior. When at their resting membrane potential, the neurons do not fire action potentials in the presence of modest sustained depolarizations, and thus are silent. In contrast, when they at their more depolarized plateau potential, even modest sustained depolarizations will trigger the repeated firing of action potentials. Compare the firing pattern shown in Figure 20.4(b) to the one presented in Figure 20.8. The cells producing the bursts in Figure 20.8 possess plateau potentials; their membrane potentials remain elevated after each action potential. This situation differs from the one depicted in Figure 20.4 in which the potential returns to values close to the resting potential after each action potential.
Last, referring again to Table 20.4 post inhibitory rebound refers to the paradoxical situation that inhibition can actually promote excitability. Some ion channels require hyperpolarization to de-inactivate and others need hyperpolarization in order to activate. If a neuron expressing these ion channels is subjected to a strong hyperpolarization that is quickly terminated, its membrane can rapidly rebound, depolarizing and firing an action potential.
20.12 Neural Circuits Are Connected to Other Circuits and Form Systems
Central pattern generators do not work in isolation. Rather, they receive inputs from sensory and higher information-processing areas and send outputs to motor neurons controlling muscles. In situations where multiple circuits must work together to generate motor rhythms, the CPGs communicate laterally to other CPGs. Properties of a typical mammalian network such as the convergence of multiple input signals and feedback control are listed in Table 20.4. The human respiratory system provides an excellent example of both of these properties.
In humans, neurons organized into a CPG in a section of the medulla called the Prebötzinger complex generate respiratory rhythms. This CPG receives input from a variety of sources denoting emotional, sleep-related, environmental, and motor activity states. The output from the CPGs drives pools of motoneurons controlling the respiratory muscles—the diaphragm, and the intercostal and abdominal muscles—that pump air in and out of the lungs. The degree of resistance to airflow is regulated by smooth muscle in the bronchial walls, and by muscles controlling the diameter of the upper airways, namely, the laryngeal, pharyngeal, and hyperglossal muscles.
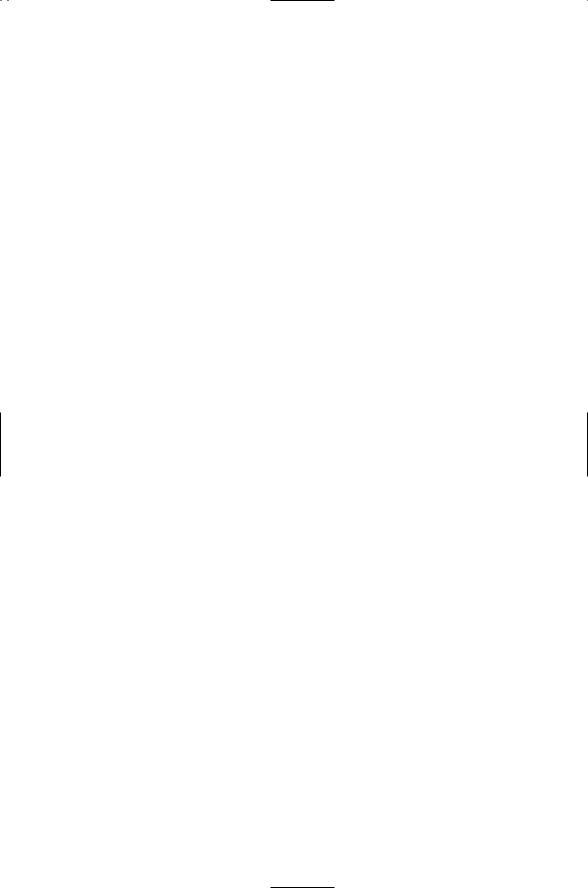
20.13 A Variety of Neuromodulators Regulate Operation |
505 |
The respiratory system is a feedback control system. Respiratory neurons send output to the diaphram, intercostal muscles, abdominal muscles, and accessory muscles of respiration. Mechanoreceptors supply feedback information on the effect of the firing patterns on the muscles. Information is supplied by stretch receptors, located in the bronchial smooth muscle, by a variety of irritant receptors located in the airway epithelium, and by C-fiber receptors located within the walls of the alveoli. Information on dissolved gases is provided by peripheral (arterial) oxygen and carbon dioxide receptors, and by central carbon dioxide (acidification) medullary receptors that monitor the cerebrospinal fluid (CSF).
Several different patterns can be generated by the respiratory CPG. Under normal breathing conditions the CPG generates a fairly regular, eupneic firing pattern. However, under conditions of oxygen starvation/ hypoxia, the patterns generated within the CPG will change to either a sighing rhythm or to a gasping rhythm. One of the features that characterize neurons lying within the CPG is the presence of neurokinin-1 receptors, the receptors for the peptide neuromodulator, substance P. The neurons expressing these receptors not only generate respiratory rhythms, but also alter their firing frequency in response to the presence or absence of substance P and other neuromodulators that, in turn, reflect oxygen status and other types of regulatory information.
20.13 A Variety of Neuromodulators Regulate
Operation of the Crustacean STG
The neurons that make up the crustacean stomatogastric ganglion express receptors for a number of amine and crustacean-specific peptide (CSP) neuromodulators. Receptors are present for (amines) serotonin, dopamine, and octopamine, and for (CSPs) crustacean cardioactive peptide (CCAP), proctolin, and cholecystokinin (CCK). These neuromodulators not only alter the firing patterns of the central pattern generators, but also can trigger aggressive behavior. Most of the CSPs are released by neurons that send their axons to the STG. The usual effect of the peptides is to initiate one or more of the rhythms—gastric, pyloric, and cardiac sac. In some instances, a neuropeptide such as red pigment-concentrating hormone (RPCH) triggers the functional reconfiguration of the circuits. The reconfiguration may take the form of circuit fusion or appear as alterations in circuit membership. In the former case, two or more circuits may fuse together so that they work together rather than independently. In the latter instance, a neuron, previously firing as part of one circuit may switch its firing properties and become part of another circuit.
The amine neuromodulators act on the ion channels, enhancing some currents and inhibiting others. They exert their influences by either shifting the activation and or deactivation curves or by increasing the maximal con-
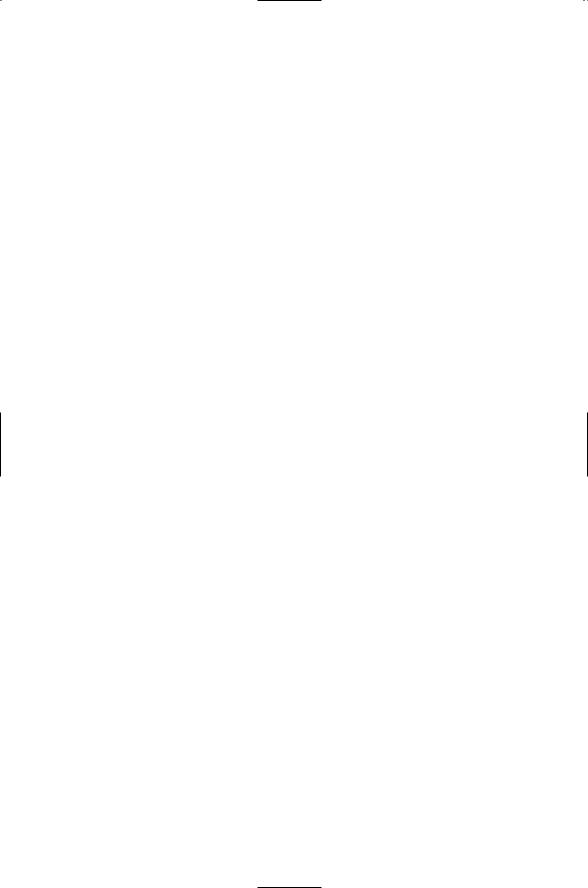
506 20. Neural Rhythms
ductances for the ion channels. These changes lead to alterations in phase of one neuron relative to others within the CPG and change the number of action potentials generated in a given time interval.
As indicated in Table 20.4, neuromodulatory signals can originate either within a CPG or externally to it. There are many sources of external inputs in the central nervous system. In vertebrates these sources are organized into centers that project out to, and make contact with, neurons in most areas of the brain. These centers are able to influence the response properties of many circuits simultaneously. Intrinsic modulation is far more specific in its actions. In the stomatogastric ganglion, modulatory inputs are supplied by higher brain centers and by feedback from sensory neurons. These modulatory inputs are sensitive to the outputs of the circuit being modified and are local to that circuit. These influence the rhythms being produced and the attendant behaviors.
20.14 Motor Systems Adapt to Their Environment and Learn
The nudibranch gastropod Tritonia diomedea is preyed upon by starfish. When Tritonia comes into contact with a foot of a starfish, it changes its behavior from a slow-moving feeding mode to a fast-moving escape swimming mode. The circuitry that makes this escape response possible includes, besides the sensory neurons, a command interneuron that receives sensory information, and a central pattern generator that receives information from the command dorsal ramp interneuron (DRI) to start the escape swim rhythm. The CPG contains three kinds of interneurons—an interneuron called C2, a pair of ventral swim interneurons (VSIs), and three dorsal swim interneurons (DSIs). The output from the CPG is sent to an array of left and right flexion motor neurons that drive the muscles responsible for escape swimming (Figure 20.9).
In Tritonia, the swim CPG produces either a swimming rhythm or a nonswimming withdrawal response. The transformation of nonswimming into swimming rhythms involves modulatory signaling by serotonin. The command interneuron sends swim signals to the DSIs. In response to these initiating signals, the DSIs release serotonin, which binds to receptors on the C2 neuron. In response to serotonin binding, the C2 interneuron increases the amount of neurotransmitter released from its presynaptic terminals and exhibits an increased excitability. The effect of the increased neurotransmitter release is to strengthen the synaptic connection from the C2 interneuron back to the DSIs thereby increasing the DSIs excitability through this positive feedback loop. (In the absence of the increased neurotransmitter release, the DSIs are repressed by inhibitory signaling between themselves). The increased excitability of the C2 neuron enables it to trigger and maintain the DSIs’ swim rhythm. If the firing rate of the C2 neuron falls below the swim threshold, escape swimming will not occur. In the terminology of
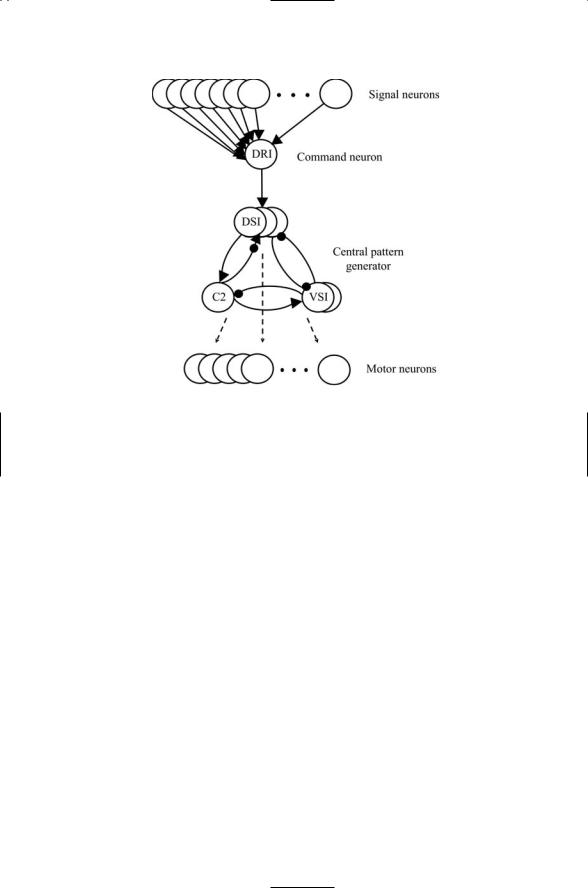
20.14 Motor Systems Adapt to Their Environment and Learn |
507 |
FIGURE 20.9. Tritonia escape circuit: Upstream signals conveyed by a set of about 80 S cells are relayed to the command neuron, the dorsal ramp interneuron (DRI), which sends its synaptic output to a set of three dorsal swim interneurons (DSIs) belonging to the central pattern generator. The CPG contains, besides the DSIs, a pair of ventral swim interneurons (VSIs) and a single cerebral cell 2 (C2) neuron. The three kinds of CPG neurons make synaptic contacts with a set of about 55 dorsal and ventral flexion motor neurons. In the diagram, sharp arrows denote excitatory connections; filled circles represent inhibitory connections, and the hybrid arrow/circle symbol denotes a multiple component (excitatory and inhibitory) monosynaptic contact.
Table 20.4, the alteration in the firing pattern of C2 by serotonin released by the DSIs is an example of intrinsic neuromodulation.
The serotonin-mediated changes in rhythmicity are long-lasting in that they outlast the stimuli that produced them. When the serotonin molecules bind to GPCRs on the postsynaptic cell, they activate Gi alpha subunits of the heterotrimeric G proteins docked nearby. These subunits stimulate adenylyl cyclase to increase its synthesis of cAMP second messenger molecules leading to alterations in the way the ion channels operate and the cell responds to further stimuli. The organism will exhibit an increased responsiveness to further noxious stimuli and a reduced responsiveness to harmless ones. The process of more rapidly responding to the dangerous stimuli is called sensitization, and the process of responding less strongly to benign stimuli is known as habituation. Both types of adaptive change are examples of learning and have been extensively studied in the snails, crustaceans, and leech.
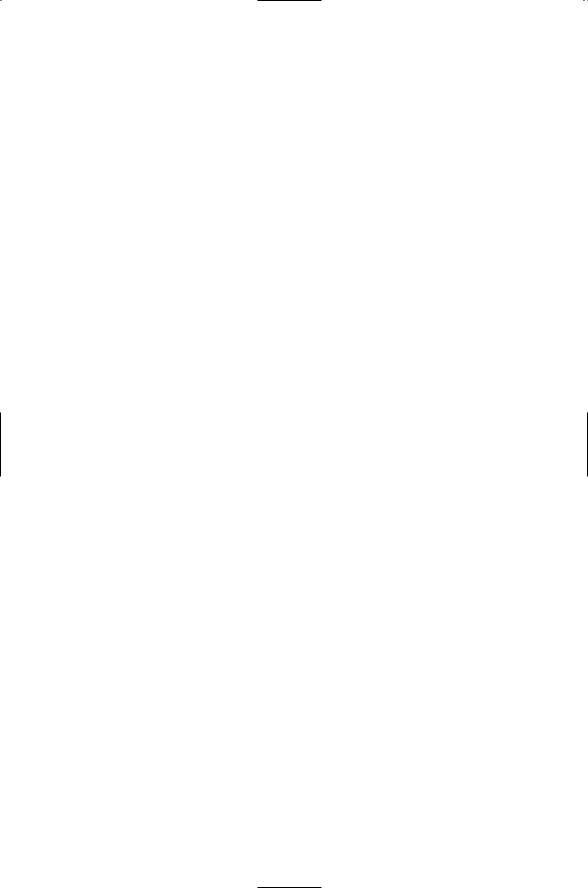
508 20. Neural Rhythms
References and Further Reading
General References
Hille B [1992]. Ionic Channels of Excitable Membranes (2nd edition). Sunderland MA: Sinauer Associates, Inc.
Kaczmarek LK, and Levitan IB [1987]. Neuromodulation. New York: Oxford University Press, Inc.
Llinás RR [1988]. The intrinsic electrophysiological properties of mammalian neurons: Insights into central nervous system function. Science, 242: 1654–1664.
Nicholls JG, Wallace BG, Fuchs PA, and Martin AR [2001]. From Neuron to Brain: A Cellular Approach to the Function of the Nervous System (4th edition).
Sunderland MA: Sinauer Associates, Inc.
Heartbeat
Boyett MR, Honjo H, and Kodama I [2000]. The sinoatrial node, a heterogeneous pacemaker structure. Cardiovasc. Res., 47: 658–687.
Schram G, et al. [2002]. Differential distribution of cardiac ion channel expression as a basis for regional specialization in electrical function. Circ. Res., 90: 939–950.
HCN Channels
DiFrancesco D [1999]. Dual allosteric modulation of pacemaker (f) channels by cAMP and voltage in rabbit SA node. J. Physiol., 515: 367–376.
Ludwig A, et al. [1998]. A family of hyperpolarization-activated mammalian cation channels. Nature, 393: 587–591.
Zagotta WN, et al. [2003]. Structural basis for modulation and agonist specificity of HCN pacemaker channels. Nature, 425: 200–205.
Thalamocortical Rhythms
Huguenard JR, and Prince DA [1992]. A novel T-type current underlies prolonged Ca2+ dependent burst firing in GABAergic neurons of rat thalamic reticular nucleus. J. Neurosci., 12: 3804–3817.
McCormick DA, and Huguenard JR [1992]. A model of the electrophysiological properties of thalamocortical relay neurons. J. Neurophysiol., 68: 1384–1400.
Munk MHJ, et al. [1996]. Role of reticular activation in the modulation of intracortical synchronization. Science, 272: 271–274.
Steriade M, Amzica F, and Contreras D [1996]. Sunchronization of fast (30–40 Hz) spontaneous cortical rhythms during brain activation. J. Neurosci., 16: 392–417.
Steriade M, McCormick DA, and Sejnowski TJ [1993]. Thalamocortical oscillations in the sleeping and awake brain. Science, 262: 679–685.
von Krosigk M, Bal T, and McCormick DA [1993]. Cellular mechanisms of a synchronized oscillation in the thalamus. Science, 261: 361–364.
Epilepsy
Crunelli V, and Leresche N [2002]. Childhood absence epilepsy: genes, channels, neurons and networks. Nature Rev. Neurosci., 3: 371–382.
Velazquez JPL, and Carlen PL [2000]. Gap junctions, synchrony and seizures. Trends Neurosci., 23: 68–74.
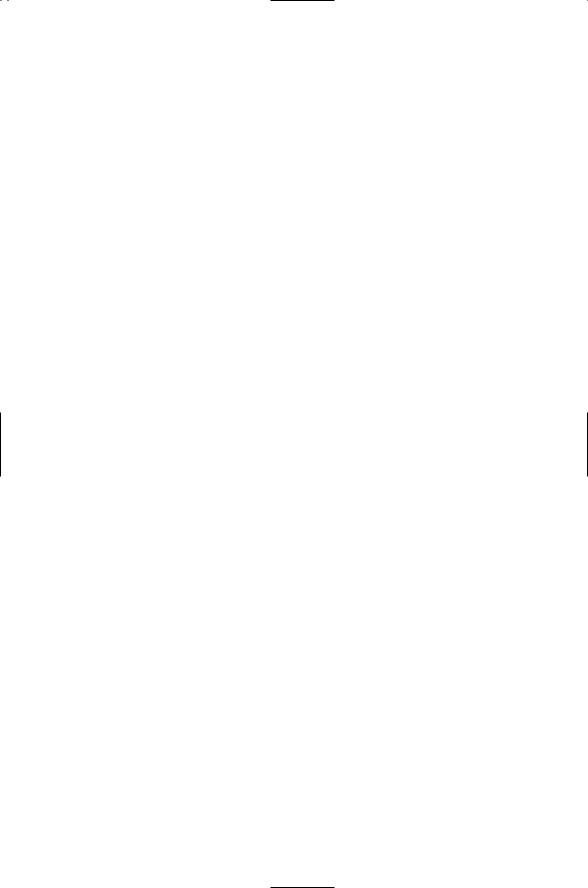
References and Further Reading |
509 |
Gap Junctions
Bruzzone R, White TW, and Paul DL [1996]. Connections with connexins: The molecular basis of direct intercellular signaling. Eur. J. Biochem., 238: 1–27.
Evans WH, and Martin PEM [2002]. Gap junctions: Structure and function. Mol. Mem. Biol., 19: 121–136.
Kumar NM, and Gilula NB [1996]. The gap junction communication channel. Cell, 84: 381–388.
Simon AM, and Goodenough DA [1998]. Diverse functions of vertebrate gap junctions. Trends Cell Biol., 8: 477–483.
Unger VM, et al. [1999]. Three-dimensional structure of a recombinant gap junction membrane channel. Science, 283: 1176–1180.
GABAergic Neurons and Gap Junction-Mediated Synchrony
Galarreta M, and Hestrin S [2001]. Electrical synapses between GABA-releasing interneurons. Nature Rev. Neurosci., 2: 425–433.
Galarreta M, and Hestrin S [1999]. A network of fast-spiking cells in the neocortex connected by electrical synapses. Nature, 402: 72–75.
Gibson JR, Belerlein M, and Connors BW [1999]. Two networks of electrically coupled inhibitory neurons in neocortex. Nature, 402: 75–79.
Tamás G, et al. [2000]. Proximally targeted GABAergic synapses and gap junctions synchronize cortical interneurons. Nature Neurosci., 3: 366–371.
Central Pattern Generators
Grillner S, et al. [1998]. Intrinsic function of a neuronal network—A vertebrate central pattern generator. Brain Res. Revs., 26: 184–197.
Grillner S, et al. [1995]. Neural networks that co-ordinate locomotion and body orientation in lamphrey. Trends. Neurosci., 18: 270–279.
Marder E, and Bucher D [2001]. Central pattern generators and the control of rhythmic movement. Curr. Biol., 11: R986–R996.
Marder E, and Calabrese RL [1996]. Principles of rhythmic motor pattern generation. Physiol. Rev., 76: 687–717.
Nusbaum MP, and Beenhakker MP [2002]. A small-systems approach to motor pattern generation. Nature, 417: 343–350.
Respiration
Gray PA, et al. [1999]. Modulation of the respiratory frequency by peptidergic input to the rhythmogenic neurons in the Prebötzinger complex. Science, 286: 1566– 1568.
Lieske SP, et al. [2000]. Reconfiguration of the neural network controlling multiple breathing patterns: Eupnea, sighs and gasps. Nature Neurosci., 3: 600–607.
Ramirez JM, and Richter DW [1996]. The neuronal mechanisms of respiratory rhythm generation. Curr. Opin. Neurobiol., 6: 817–825.
Motor Learning and Memory
Kandel ER [2000]. The molecular biology of memory storage: A dialogue between genes and synapses. Science, 294: 1030–1038.
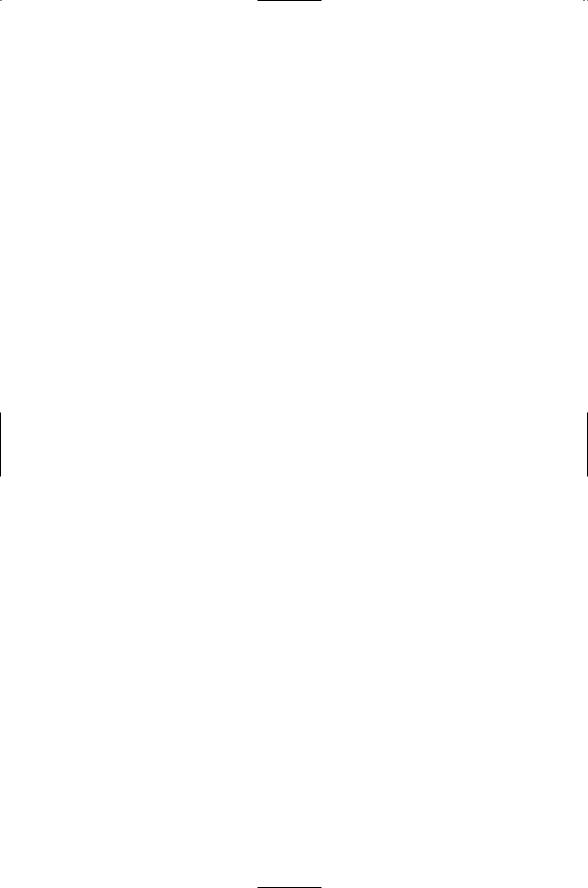
510 20. Neural Rhythms
Problems
20.1 A small number of ion channels have particularly prominent roles in generating rhythmic patterns of action potentials. One of these, the HCN channel, was discussed in Section 20.2 while another, the T-type calcium channel, was discussed in Section 20.4. Make a sketch of the activation and deactivation functions for the T-type calcium channel and the sodium channels responsible for action potential upstrokes.
Recall from the discussion in Section 20.4 that at the resting potential the T-type calcium channel is activating while the sodium channel is deactivating. In the plots of current versus membrane potential, indicate the location of a resting potential and position the four curves so they satisfy the relationship just mentioned.
20.2 The utility of a gradually depolarizing membrane potential was discussed in Section 20.11 and illustrated in Figure 20.1. As noted in that discussion, this behavior greatly enhances excitability in cells possessing plateau potentials. When two cells of this type are coupled through mutual inhibition they can synchronize their firing acting as oscillatory units that produce motor rhythms. List some types of currents that promote gradual declining membrane potentials of the sort depicted in the figure.

21
Learning and Memory
The learning and memory formation processes carried out by the snails and other invertebrates is certainly more modest than that of humans, yet the two are similar. Each involve alterations in behavioral responses to stimuli brought on by experience. Learning is the adaptive process whereby changes in behavior are induced in response to experience. Memory is the record, or trace, underlying the changes in behavior. The records in all organisms, snails and mice, flies and humans, are stored in the patterns of connection between neurons. During learning and memory formation some connections are strengthened while others are weakened. For instance, in the case of sensitization discussed at the end of the last chapter, connections between neurons were strengthened. This led to behavioral changes. The snails were able to respond with increased rapidity to noxious stimuli.
The term efficiency of synaptic transmission refers to the magnitude of the response generated in a postsynaptic neuron when an action potential is generated in the presynaptic neuron. In many situations, the efficiency of the transmission is not fixed but instead varies in ways that depend on use. The changes in efficiency can be transient, lasting for a few milliseconds, or can be permanent, lasting for a lifetime. Connections may be strengthened or be weakened or disappear altogether. Synaptic plasticity is the term used to describe the use-dependent, or adaptive, changes in the efficiency of synaptic transmission between preand postsynaptic cells.
The ability to vary connection strengths is an important one. It allows the nervous system to form its precise connections and neural circuits during development; it gives rise to a unique personality for each individual; and it makes possible learning throughout life. Cortical circuits develop over time in several stages. The first stage of circuit development involves neurite outgrowth, growth cone pathfinding and then synaptogenesis. As the circuits become functional and can respond to synaptic input, ineffective and improper connections are pruned, and neurons that are no longer needed die off through programmed cell death. The process of varying the strengths of synaptic connections continues throughout life. It underlies the development of simple motor skills as well as highly coordinated eye and hand
511