
- •Contents
- •Contributors
- •Preface
- •1 Introduction, with the biological basis for cell mechanics
- •Introduction
- •The role of cell mechanics in biological function
- •Maintenance of cell shape
- •Cell migration
- •Mechanosensing
- •Stress responses and the role of mechanical forces in disease
- •Active cell contraction
- •Structural anatomy of a cell
- •The extracellular matrix and its attachment to cells
- •Transmission of force to the cytoskeleton and the role of the lipid bilayer
- •Intracellular structures
- •Overview
- •References
- •2 Experimental measurements of intracellular mechanics
- •Introduction
- •Forces to which cells are exposed in a biological context
- •Methods to measure intracellular rheology by macrorheology, diffusion, and sedimentation
- •Whole cell aggregates
- •Sedimentation of particles
- •Diffusion
- •Mechanical indentation of the cell surface
- •Glass microneedles
- •Cell poker
- •Atomic force microscopy
- •Mechanical tension applied to the cell membrane
- •Shearing and compression between microplates
- •Optical traps
- •Magnetic methods
- •Twisting of magnetized particles on the cell surface and interior
- •Passive microrheology
- •Optically detected individual probes
- •One-particle method
- •Two-particle methods
- •Dynamic light scattering and diffusing wave spectroscopy
- •Fluorescence correlation spectroscopy
- •Optical stretcher
- •Acoustic microscopy
- •Outstanding issues and future directions
- •References
- •3 The cytoskeleton as a soft glassy material
- •Introduction
- •Magnetic Twisting Cytometry (MTC)
- •Measurements of cell mechanics
- •The structural damping equation
- •Reduction of variables
- •Universality
- •Scaling the data
- •Collapse onto master curves
- •Theory of soft glassy rheology
- •What are soft glassy materials
- •Sollich’s theory of SGMs
- •Soft glassy rheology and structural damping
- •Open questions
- •Biological insights from SGR theory
- •Malleability of airway smooth muscle
- •Conclusion
- •References
- •4 Continuum elastic or viscoelastic models for the cell
- •Introduction
- •Purpose of continuum models
- •Principles of continuum models
- •Boundary conditions
- •Mechanical and material characteristics
- •Example of studied cell types
- •Blood cells: leukocytes and erythrocytes
- •Limitations of continuum model
- •Conclusion
- •References
- •5 Multiphasic models of cell mechanics
- •Introduction
- •Biphasic poroviscoelastic models of cell mechanics
- •Analysis of cell mechanical tests
- •Micropipette aspiration
- •Cells
- •Biphasic properties of the pericellular matrix
- •Indentation studies of cell multiphasic properties
- •Analysis of cell–matrix interactions using multiphasic models
- •Summary
- •References
- •6 Models of cytoskeletal mechanics based on tensegrity
- •Introduction
- •The cellular tensegrity model
- •The cellular tensegrity model
- •Do living cells behave as predicted by the tensegrity model?
- •Circumstantial evidence
- •Prestress-induced stiffening
- •Action at a distance
- •Do microtubules carry compression?
- •Summary
- •Examples of mathematical models of the cytoskeleton based on tensegrity
- •The cortical membrane model
- •Tensed cable nets
- •Cable-and-strut model
- •Summary
- •Tensegrity and cellular dynamics
- •Conclusion
- •Acknowledgement
- •References
- •7 Cells, gels, and mechanics
- •Introduction
- •Problems with the aqueous-solution-based paradigm
- •Cells as gels
- •Cell dynamics
- •Gels and motion
- •Secretion
- •Muscle contraction
- •Conclusion
- •Acknowledgement
- •References
- •8 Polymer-based models of cytoskeletal networks
- •Introduction
- •The worm-like chain model
- •Force-extension of single chains
- •Dynamics of single chains
- •Network elasticity
- •Nonlinear response
- •Discussion
- •References
- •9 Cell dynamics and the actin cytoskeleton
- •Introduction: The role of actin in the cell
- •Interaction of the cell cytoskeleton with the outside environment
- •The role of cytoskeletal structure
- •Actin mechanics
- •Actin dynamics
- •The emergence of actin dynamics
- •The intrinsic dynamics of actin
- •Regulation of dynamics by actin-binding proteins
- •Capping protein: ‘decommissioning’ the old
- •Gelsolin: rapid remodeling in one or two steps
- •β4-thymosin: accounting (sometimes) for the other half
- •Dynamic actin in crawling cells
- •Actin in the leading edge
- •Monomer recycling: the other ‘actin dynamics’
- •The biophysics of actin-based pushing
- •Conclusion
- •Acknowledgements
- •References
- •10 Active cellular protrusion: continuum theories and models
- •Cellular protrusion: the standard cartoon
- •The RIF formalism
- •Mass conservation
- •Momentum conservation
- •Boundary conditions
- •Cytoskeletal theories of cellular protrusion
- •Network–membrane interactions
- •Network dynamics near the membrane
- •Special cases of network–membrane interaction: polymerization force, brownian and motor ratchets
- •Network–network interactions
- •Network dynamics with swelling
- •Other theories of protrusion
- •Numerical implementation of the RIF formalism
- •An example of cellular protrusion
- •Protrusion driven by membrane–cytoskeleton repulsion
- •Protrusion driven by cytoskeletal swelling
- •Discussion
- •Conclusions
- •References
- •11 Summary
- •References
- •Index
180 J.L. McGrath and C.F. Dewey, Jr.
This scaling can be compared to the results derived from semiflexible polymers with crosslinking. The latest results in this field are discussed in detail in Chapter 8. Published theories by MacKintosh and colleagues (Gittes and MacKintosh, 1998; MacKintosh et al., 1995; Chapter 8) show that the shear modulus G for densely cross-linked gels scales as G (ρ /ρs )5/2. Although the power law is similar, the value of G depends on the “entanglement length,” which is measured by thermal fluctuations of the filaments. It seems plausible that for open structures for which the typical dimensions are lp l t the thermal agitation would not play a strong role and the so-called enthalpic contribution would be negligible. From the point of view of existing experimental data taken in dilute solutions (Janmey et al., 1991),
it is very difficult to decide between the effective modulus scaling as (ρ /ρs )2 or (ρ /ρs )5/2.
Two common features between the models are important. First, the elastic modulus is independent of frequency, at least for times over which the cross-links do not turn over and for which the frequencies are sufficiently low so as to not change the basic mechanisms of deformation of the filaments. Second, the existence of cross-linking is crucial; without cross-linking, the effective modulus would be substantially lower than the observed values.
A practical consequence of the predictions of the theory is that a drop in the fraction of actin polymerized can have a very profound effect on the rigidity of the cell. In separate experiments, we have observed a drop by factors of two to three in the fraction of actin polymerized following changes from a packed monolayer to freely crawling cells (McGrath et al., 2000b; McGrath et al., 1998b). We therefore find that the freely crawling cells have a much lower effective modulus and a higher motility. This has potential implications for wound healing, endothelialization of graft materials, and the integrity of monolayers subjected to fluid-flow forces.
We have examined the theory and observations relative to the internal cytoskeleton of a static collection of cells. Cells are in a dynamic state, with the internal cytoskeleton changing continuously. The following section explores the interesting dynamics that occur within the cell and presents quantitative models for the processes that are at work.
Actin dynamics
Abundant, essential, and discovered more than a half-century ago, actin is one of the most studied of all the proteins. Many investigations have focused on the dynamic character of actin, leading to a rich quantitative understanding of actin assembly and disassembly. In this section we briefly review this history and summarize the modern understanding of actin dynamics and its regulation by key binding proteins.
The emergence of actin dynamics
The appreciation that actin has both dynamic and mechanical properties can be traced to the work of its discoverer, F.B. Straub (Mommaerts, 1992). Trying to understand the difference between a highly viscous mixture of ‘myosin B’ and a less viscous
Cell dynamics and the actin cytoskeleton |
181 |
mixture of ‘myosin A,’ Straub discovered these were not different myosins at all, but that the myosin B preparations were ‘contaminated’ by another protein that ACTivated myosIN to make the viscous solution (Feuer et al., 1948). Straub’s laboratory later revealed that the contaminating actin could itself convert between lowand highviscosity solutions with the introduction of physiological salts and/or ATP (Straub and Feuer, 1950). Further data from Straub demonstrated that the phase change occurred because a globular protein (‘G-actin’) polymerized into long filaments (‘F-actin’) and that the filamentous form could hydrolyze ATP. With the discovery of sarcomere structure (Huxley and Hanson, 1954) and the sliding filament model of muscle contraction in the 1950’s (Huxley, 1957), actin’s role as the structural thin filament of muscle was in place. The significance of actin’s dynamic properties, however, remained unclear.
Over the next several decades actin was identified in every eukaryotic cell investigated. Extracts formed from macrophages (Stossel and Hartwig, 1976b) or ananthamoeba (Pollard and Ito, 1970) could be made to ‘gel’ in a manner that involved actin polymerization. Actin filaments were found to be concentrated near ruffling membranes and in fibroblast ‘stress fibers’ (Goldman et al., 1975; Lazarides and Weber, 1974), and cell movements could be halted with the actin-specific cytochalasins (Carter, 1967). Cytochalasins were found to block actin polymerization (Brenner and Korn, 1979), to inhibit the gelation of extracts (Hartwig and Stossel, 1976; Stossel and Hartwig, 1976; Weihing, 1976), and to reduce the strengths of reconstituted actin networks (Hartwig and Stossel, 1979). With these findings, actin’s role as a structural protein appeared universal. However, unlike in muscle cells, actin’s dynamic properties allow nonmuscle cells to tailor diverse and dynamic mechanical structures. Elucidating the intrinsic and regulated dynamics of actin was rightly viewed as a fundamental question in cell physiology, and became a lifelong pursuit for many talented biologists and physicists.
The intrinsic dynamics of actin
The pioneering work on protein self-assembly by Fumio Oosawa (see Fig. 9-6) led to some of the earliest insights into the process of actin polymerization. Using light scattering to follow the progress of polymerization, Oosawa noticed that actin polymerization typically began several minutes after the addition of polymer-inducing salts. He concluded that during the early ‘lag’ phase of polymerization, monomers form nuclei in an unfavorable reaction, but that once formed, nuclei are rapidly stabilized by monomer addition (Oosawa and Kasai, 1962). Because the addition of monomers to filaments is a highly favorable reaction, the lag phase could be overcome by adding preformed filaments as nuclei. Oosawa’s studies indicated that the nucleus was formed of three monomers, a number that has stood further scrutiny (Wegner and Engel, 1975).
Oosawa described the mechanism of actin assembly by constructing some of the first kinetic models of the process (Oosawa and Asakura, 1975). Because experiments revealed actin filaments to have biochemically distinct ‘barbed’ and ‘pointed’ ends (Woodrum et al., 1975), and because ATPand ADP-bound monomers are capable of assembling at these ends, Oozawa considered four assembly and four disassembly
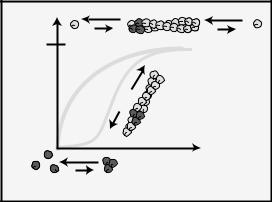
182 J.L. McGrath and C.F. Dewey, Jr.
treadmilling
100%
% actin merizedylop
|
|
|
|
|
|
|
|
s |
|
|
|
|
|
|
|
|
d |
|
|
|
|
|
|
|
|
e |
|
|
|
|
|
|
|
|
e |
|
|
|
|
|
|
|
|
s |
|
|
|
|
|
|
|
|
|
t |
|
|
|
|
barbed |
|
|
|
|
n |
|
|
|
|
|
|
|
|
|
e |
|
|
|
|
|
f |
i |
l |
a |
m |
|
|
|
|
|
|
|
|
|
|
|
||||
|
|
|
|
|
|
|
|
||
|
|
|
|
|
|
|
|
|
pointed
time
nucleation 'lag'
Fig. 9-6. Intrinsic actin dynamics. Oosawa and colleagues found that actin polymerizes in two phases:
(a) an unfavorable nucleation phase involving an actin trimer and (b) a rapid assembly phase in which actin monomers add to both barbed and pointed ends. In the presence of excess ATP, actin does not polymerize to equilibrium but to a ‘treadmilling’ steady-state in which monomers continuously add to the barbed end and fall off of the pointed end.
reactions. Oozawa also assumed that spontaneous filament fragmentation and annealing ultimately determined the number of filaments in solution; this was later validated with detailed modeling (Murphy et al., 1988; Sept et al., 1999).
Obtaining reliable constants for the assembly and disassembly rates in the Oosawa model was a challenge that would occupy many years. Indirect assays gave conflicting results because of their inability to control or accurately measure the number of filaments in solutions. In 1986, Pollard’s direct electron microscopic measurements of growth on individual nuclei produced numbers that are now the most widely cited (Pollard, 1986).
A decade before Pollard’s experiments, Albert Wegner proposed that actin assembly does not proceed to equilibrium, but to a steady-state in which assembled subunits continuously traverse filaments from the barbed to the pointed end (Wegner and Engel, 1975). His now-classic experiments revealed that even after bulk polymerization had halted, filaments continued to hydrolyze ATP. Wegner decided that the results could only be explained if ATP-carrying monomers had a higher affinity for barbed ends than for pointed ends, and that the energy of ATP hydrolysis must be used to sustain the imbalance. Wegner suggested that, on average, ATP-G-actin assembles at barbed ends, hydrolyzes ATP in the filament interior, and disassembles bound to ADP at pointed ends. Thus Wegner was the first to introduce the concept of actin filament ‘treadmilling,’ although the term itself must be credited to Kirschner some years later (Kirschner, 1980). While Pollard’s rate constants were not measured at steady-state, they clearly suggested barbed ends had a higher affinity for monomer in the presence of ATP and thus supported the existence of treadmilling.
A difficulty with both the Pollard and the Wegner studies was the lack of an accurate measure of the ATP hydrolysis rate on F-actin. Within the same year of Pollard’s paper, Carlier and colleagues reported that ATP hydrolysis occurred less than a second after subunit addition (Carlier et al., 1987). Because this time scale for ATP hydrolysis