
- •Contents
- •Contributors
- •Preface
- •1 Introduction, with the biological basis for cell mechanics
- •Introduction
- •The role of cell mechanics in biological function
- •Maintenance of cell shape
- •Cell migration
- •Mechanosensing
- •Stress responses and the role of mechanical forces in disease
- •Active cell contraction
- •Structural anatomy of a cell
- •The extracellular matrix and its attachment to cells
- •Transmission of force to the cytoskeleton and the role of the lipid bilayer
- •Intracellular structures
- •Overview
- •References
- •2 Experimental measurements of intracellular mechanics
- •Introduction
- •Forces to which cells are exposed in a biological context
- •Methods to measure intracellular rheology by macrorheology, diffusion, and sedimentation
- •Whole cell aggregates
- •Sedimentation of particles
- •Diffusion
- •Mechanical indentation of the cell surface
- •Glass microneedles
- •Cell poker
- •Atomic force microscopy
- •Mechanical tension applied to the cell membrane
- •Shearing and compression between microplates
- •Optical traps
- •Magnetic methods
- •Twisting of magnetized particles on the cell surface and interior
- •Passive microrheology
- •Optically detected individual probes
- •One-particle method
- •Two-particle methods
- •Dynamic light scattering and diffusing wave spectroscopy
- •Fluorescence correlation spectroscopy
- •Optical stretcher
- •Acoustic microscopy
- •Outstanding issues and future directions
- •References
- •3 The cytoskeleton as a soft glassy material
- •Introduction
- •Magnetic Twisting Cytometry (MTC)
- •Measurements of cell mechanics
- •The structural damping equation
- •Reduction of variables
- •Universality
- •Scaling the data
- •Collapse onto master curves
- •Theory of soft glassy rheology
- •What are soft glassy materials
- •Sollich’s theory of SGMs
- •Soft glassy rheology and structural damping
- •Open questions
- •Biological insights from SGR theory
- •Malleability of airway smooth muscle
- •Conclusion
- •References
- •4 Continuum elastic or viscoelastic models for the cell
- •Introduction
- •Purpose of continuum models
- •Principles of continuum models
- •Boundary conditions
- •Mechanical and material characteristics
- •Example of studied cell types
- •Blood cells: leukocytes and erythrocytes
- •Limitations of continuum model
- •Conclusion
- •References
- •5 Multiphasic models of cell mechanics
- •Introduction
- •Biphasic poroviscoelastic models of cell mechanics
- •Analysis of cell mechanical tests
- •Micropipette aspiration
- •Cells
- •Biphasic properties of the pericellular matrix
- •Indentation studies of cell multiphasic properties
- •Analysis of cell–matrix interactions using multiphasic models
- •Summary
- •References
- •6 Models of cytoskeletal mechanics based on tensegrity
- •Introduction
- •The cellular tensegrity model
- •The cellular tensegrity model
- •Do living cells behave as predicted by the tensegrity model?
- •Circumstantial evidence
- •Prestress-induced stiffening
- •Action at a distance
- •Do microtubules carry compression?
- •Summary
- •Examples of mathematical models of the cytoskeleton based on tensegrity
- •The cortical membrane model
- •Tensed cable nets
- •Cable-and-strut model
- •Summary
- •Tensegrity and cellular dynamics
- •Conclusion
- •Acknowledgement
- •References
- •7 Cells, gels, and mechanics
- •Introduction
- •Problems with the aqueous-solution-based paradigm
- •Cells as gels
- •Cell dynamics
- •Gels and motion
- •Secretion
- •Muscle contraction
- •Conclusion
- •Acknowledgement
- •References
- •8 Polymer-based models of cytoskeletal networks
- •Introduction
- •The worm-like chain model
- •Force-extension of single chains
- •Dynamics of single chains
- •Network elasticity
- •Nonlinear response
- •Discussion
- •References
- •9 Cell dynamics and the actin cytoskeleton
- •Introduction: The role of actin in the cell
- •Interaction of the cell cytoskeleton with the outside environment
- •The role of cytoskeletal structure
- •Actin mechanics
- •Actin dynamics
- •The emergence of actin dynamics
- •The intrinsic dynamics of actin
- •Regulation of dynamics by actin-binding proteins
- •Capping protein: ‘decommissioning’ the old
- •Gelsolin: rapid remodeling in one or two steps
- •β4-thymosin: accounting (sometimes) for the other half
- •Dynamic actin in crawling cells
- •Actin in the leading edge
- •Monomer recycling: the other ‘actin dynamics’
- •The biophysics of actin-based pushing
- •Conclusion
- •Acknowledgements
- •References
- •10 Active cellular protrusion: continuum theories and models
- •Cellular protrusion: the standard cartoon
- •The RIF formalism
- •Mass conservation
- •Momentum conservation
- •Boundary conditions
- •Cytoskeletal theories of cellular protrusion
- •Network–membrane interactions
- •Network dynamics near the membrane
- •Special cases of network–membrane interaction: polymerization force, brownian and motor ratchets
- •Network–network interactions
- •Network dynamics with swelling
- •Other theories of protrusion
- •Numerical implementation of the RIF formalism
- •An example of cellular protrusion
- •Protrusion driven by membrane–cytoskeleton repulsion
- •Protrusion driven by cytoskeletal swelling
- •Discussion
- •Conclusions
- •References
- •11 Summary
- •References
- •Index
8 Polymer-based models of cytoskeletal networks
F.C. MacKintosh
ABSTRACT: Most plant and animal cells possess a complex structure of filamentous proteins and associated proteins and enzymes for bundling, cross-linking, and active force generation. This cytoskeleton is largely responsible for cell elasticity and mechanical stability. It can also play a key role in cell locomotion. Over the last few years, the single-molecule micromechanics of many of the important constituents of the cytoskeleton have been studied in great detail by biophysical techniques such as high-resolution microscopy, scanning force microscopy, and optical tweezers. At the same time, numerous in vitro experiments aimed at understanding some of the unique mechanical and dynamic properties of solutions and networks of cytoskeletal filaments have been performed. In parallel with these experiments, theoretical models have emerged that have both served to explain many of the essential material properties of these networks, as well as to motivate quantitative experiments to determine, for example concentration dependence of shear moduli and the effects of cross-links. This chapter is devoted to theoretical models of the cytoskeleton based on polymer physics at both the level of single protein filaments and the level of solutions and networks of cross-linked or entangled filaments. We begin with a derivation of the static and dynamic properties of single cytoskeletal filaments. We then proceed to build up models of solutions and cross-linked gels of cytoskeletal filaments and we discuss the comparison of these models with a variety of experiments on in vitro model systems.
Introduction
Understanding the mechanical properties of cells and even whole tissues continues to pose significant challenges. Cells experience a variety of external stresses and forces, and they exert forces on their surroundings – for instance, in cell locomotion. The mechanical interaction of cells with their surroundings depends on structures such as cell membranes and complex networks of filamentous proteins. Although these cellular components have been known for many years, important outstanding problems remain concerning the origins and regulation of cell mechanical properties (Pollard and Cooper, 1986; Alberts et al., 1994; Boal, 2002). These mechanical factors determine how a cell maintains and modifies its shape, how it moves, and even how cells adhere to one another. Mechanical stimulus of cells can also result in changes in gene expression.
Cells exhibit rich composite structures ranging from the nanometer to the micrometer scale. These structures combine soft membranes and rather rigid filamentous
152
Polymer-based models of cytoskeletal networks |
153 |
proteins or biopolymers, among other components. Most plant and animal cells, in fact, possess a complex network structure of biopolymers and associated proteins and enzymes for bundling, cross-linking, and active force generation. This cytoskeleton is often the principal determinant of cell elasticity and mechanical stability.
Over the last few years, the single-molecule properties of many of the important building blocks of the cytoskeleton have been studied in great detail by biophysical techniques such as high-resolution microscopy, scanning force microscopy, and optical tweezers. At the same time, numerous in vitro experiments have aimed to understand some of the unique mechanical and dynamic properties of solutions and networks of cytoskeletal filaments. In parallel with these experiments, theoretical models have emerged that have served both to explain many of the essential material properties of these networks, as well as to motivate quantitative experiments to determine the way material properties are regulated by, for example, cross-linking and bundling proteins. Here, we focus on recent theoretical modeling of cytoskeletal solutions and networks.
One of the principal components of the cytoskeleton, and even one of the most prevalent proteins in the cell, is actin. This exists in both monomeric or globular (G-actin) and polymeric or filamentary (F-actin) forms. Actin filaments can form a network of entangled, branched, and/or cross-linked filaments known as the actin cortex, which is frequently found near the periphery of cells. In vivo, this network is far from passive, with both active motion and (contractile) force generation during cell locomotion, and with a strong coupling to membrane proteins that appears to play a key role in the ability of cells to sense and respond to external stresses.
In order to understand these complex structures, quantitative models are needed for the structure, interactions, and mechanical response of networks such as the actin cortex. Unlike networks and gels of most synthetic polymers, however, these networks have been clearly shown to possess properties that cannot be modeled by existing polymer theories. These properties include rather large shear moduli (compared with synthetic polymers under similar conditions), strong signatures of nonlinear response (in which, for example, the shear modulus can increase by a full factor of ten or more under modest strains of only 10 percent or so) (Janmey et al., 1994), and unique dynamics. In a very close and active collaboration between theory and experiment over the past few years, a standard model of sorts for the material properties of semiflexible polymer networks has emerged, which can explain many of the observed properties of F-actin networks, at least in vitro. Central to these models has been the semiflexible nature of the constituent filaments, which is both a fundamental property of almost any filamentous protein, as well as a clear departure from conventional polymer physics, which has focused on flexible or rod-like limits. In contrast, biopolymers such as F-actin are nearly rigid on the scale of a micrometer, while at the same time showing significant thermal fluctuations on the cellular scale of a few microns.
This chapter begins with an introduction to models of single-filament response and dynamics, and in fact, spends most of its time on a detailed understanding of these single-filament properties. Because cytoskeletal filaments are the most important structural components in cells, a quantitative understanding of their mechanical response to bending, stretching, and compression is crucial for any model of the mechanics of networks of these filaments. We shall see how these fundamental
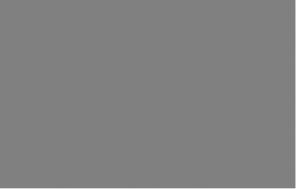
154 F.C. MacKintosh
Fig. 8-1. Entangled solution of semiflexible actin filaments. (A) In physiological conditions, individual monomeric actin proteins (G-actin) polymerize to form double-stranded helical filaments known as F-actin. These filaments exhibit a polydisperse length distribution of up to 70 µm in length. (B) A solution of 1.0 mg/ml actin filaments, approximately 0.03% of which have been labeled with rhodamine-phalloidin in order to visualize them by florescence microscopy. The average distance ξ between chains in this figure is approximately 0.3 µm. (Reprinted with permission from MacKintosh F C, K¨as J, and Janmey P A, Physical Review Letters, 75 4425 (1995). Copyright 1995 by the American Physical Society.
properties of the individual filaments can explain many of the properties of solutions and networks.
Single-filament properties
The biopolymers that make up the cytoskeleton consist of aggregates of large globular proteins that are bound together rather weakly, as compared with most synthetic, covalently bonded polymers. Nevertheless, they can be surprisingly strong. The most rigid of these are microtubules, which are hollow tube-like filaments that have a diameter of approximately 20 nm. The most basic aspect determining the mechanical behavior of cytoskeletal polymers on the cellular scale is their bending rigidity.
Even with this mechanical resistance to bending, however, cytoskeletal filaments can still exhibit significant thermally induced bending fluctuations because of Brownian motion in a liquid. Thus such filaments are said to be semiflexible or wormlike. This is illustrated in Fig. 8-1, showing fluorescently labeled F-actin filaments on the micrometer scale. The effect of the Brownian forces on the filament leads to increasingly contorted shapes over larger-length segments. The length at which significant bending fluctuations occur actually provides a simple yet quantitative characterization of the mechanical stiffness of such polymers. This thermal bending length, or persistence length p, is defined in terms of the the angular correlations (for example, of the local orientation along the polymer backbone), which decay exponentially with a characteristic length p. In simple terms, however, this just says that a typical filament in thermal equilibrium in a liquid will appear rather straight over lengths that are short compared with this persistence length, while it will begin to exhibit a random, contorted shape only on longer-length scales. The persistence lengths of a few important biopolymers are given in Table 8-1, along with their approximate diameter and length.

Polymer-based models of cytoskeletal networks |
155 |
|||
Table 8-1. Persistence lengths and other parameters of various biopolymers |
||||
(Howard, 2001; Gittes et al., 1993) |
|
|
|
|
|
|
|
|
|
|
|
|
|
|
Type |
Approximate diameter |
Persistence length |
Contour length |
|
|
|
|
|
|
DNA |
2 nm |
50 nm |
<1 m |
|
|
|
|
|
|
F-actin |
7 nm |
17 µm |
< |
|
50 µm |
||||
Microtubule |
25 nm |
1–5 mm |
10s of µm |
|
The worm-like chain model
Rigid polymers can be thought of as elastic rods, except on a small scale. The mechanical description of these is essentially the same as for a macroscopic rod with quantitative differences in parameters. The important role of thermal fluctuations, however, introduces a qualitative difference from the macroscopic case. Because the diameter of a filamentous protein is so much smaller than other length scales of interest – and especially the cellular scale – it is often sufficient to think of a filament as an idealized curve that resists bending. This is the essence of the so-called worm-like chain model. This can be described by an energy of the form,
Hbend |
|
κ |
ds |
|
∂ t 2 |
, |
(8.1) |
|||
|
= |
|
|
|
|
|
|
|
|
|
|
2 |
|
|
|
∂ s |
|
|
|
||
|
|
|
|
|
|
|
|
|
|
|
where κ is the bending modulus and t is a (unit) tangent vector along the chain. The variation (derivative) of the tangent is a measure of curvature, which appears here quadratically because it is assumed that there is no preferred direction of curvature. Here, the chain position r(s) is described in terms of a coordinate s corresponding to the length along the chain backbone. Hence, the tangent vector
t = ∂r . ∂ s
These quantities are illustrated in Fig. 8-2.
The bending modulus κ has units of energy times length. A natural energy scale for a rod subject to Brownian fluctuations is kT , where T is the temperature and k is Boltzmann’s constant. This is the typical kinetic energy of a molecule or particle. The persistence length described above is simply given by p = κ/(kT ), because the fluctuations tend to decrease with stiffness κ and increase with temperature. As noted, this is the typical length scale over which the polymer forgets its orientation, due to the constant Brownian forces it experiences in a medium at finite temperature.
More precisely, for a homogeneous rod of diameter 2a consisting of a homogeneous elastic material, the bending modulus should be proportional to the Young’s modulus E. The Young’s modulus, or the stiffness of the material, has units of energy per volume. Thus, on dimensional grounds, we expect that κ Ea4. In fact (Landau and Lifshitz 1986),
κ= π Ea4.
4
The prefactor in front of Ea4 depends on the geometry of the rod (in other words, its cross-section). The factor π a4/4 is for a solid rod of radius a. For a hollow tube, such