
Intermediate Physics for Medicine and Biology - Russell K. Hobbie & Bradley J. Roth
.pdf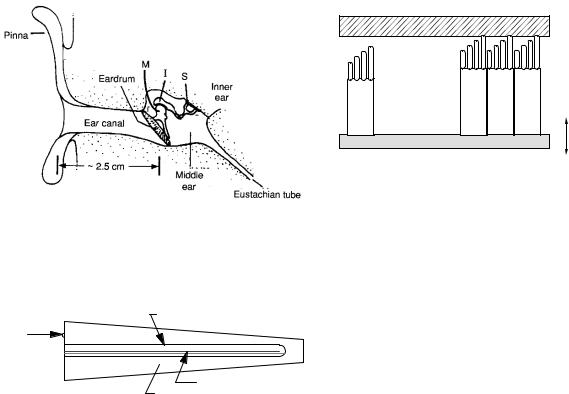

350 13. Sound and Ultrasound
is exponential. The amplitude attenuation coe cient5 is |
|
105 |
|
|
|
|
|
|
|
|
|
|||
defined by |
|
|
|
|
|
|
Lung |
|
|
|
|
|
|
|
1 dp |
|
|
|
|
|
|
|
|
|
|
|
|
||
(13.35) |
|
|
|
|
|
|
|
|
|
|
|
|
||
α = −p dx , |
-1) |
104 |
|
|
|
|
|
|
|
|
|
|||
where x is the distance the wave travels in the medium. |
m |
|
|
|
Skull |
|
|
|
|
|
|
|
||
(dB |
10 |
3 |
|
Muscle across fibers |
|
|
|
|||||||
The sound pressure amplitude decays exponentially: |
|
|
|
|
|
|||||||||
|
|
|
|
|
|
|||||||||
coefficient |
|
|
|
|
|
|
||||||||
p(x) = p(0)e−αx. |
(13.36) |
|
|
|
|
Muscle along fibers |
|
|
|
|||||
102 |
|
Liver |
|
|
|
|
|
|
|
|||||
Since the intensity is proportional to p2, |
|
|
|
|
|
|
|
|
|
|
|
|
||
|
Attenuation |
|
|
|
Blood |
|
|
|
|
|
|
|
||
|
|
101 |
|
|
|
|
|
|
|
|
||||
I(x) = I(0)e−2αx. |
(13.37) |
|
|
|
|
|
|
|
|
|
||||
|
|
|
|
|
|
|
|
|
|
|
||||
The intensity attenuation coe cient is µ = 2α. In |
100 |
|
|
|
|
|
|
|
|
|
||||
acoustics, the attenuation is usually expressed in deci- |
|
|
|
|
Water |
|
|
|
|
|
|
|
||
bels per meter, which is then independent of whether µ |
|
|
|
|
|
|
|
|
|
|
|
|||
|
10-1 |
|
|
|
|
|
|
|
|
|
||||
or α is used.6 |
|
|
6 |
2 |
3 |
4 |
5 |
6 7 |
8 9 |
|
7 |
|||
|
|
|
10 |
|
||||||||||
The wave equation for acoustics is an approximation, |
|
|
|
|
|
|
|
|
10 |
|
||||
|
|
|
|
|
Frequency (Hz) |
|
|
|
|
|||||
because the basic equations of fluid dynamics are non- |
|
|
|
|
|
|
|
|
|
|||||
|
|
|
|
|
|
|
|
|
|
|
|
|||
linear. Therefore e ects that we have ignored, such as |
FIGURE 13.11. Representative values of the attenuation co- |
|||||||||||||
waveform distortion, the generation of harmonics, and in- |
e cient for ultrasound. |
|
|
|
|
|
|
|
||||||
creased attenuation may occur, particularly at high sound |
|
|
|
|
|
|
|
|
|
|
|
|
||
intensities. |
|
13.7 Medical Uses of Ultrasound |
|
|
||||||||||
In air, the attenuation depends on the frequency of the |
|
|
||||||||||||
sound and the temperature and humidity of the air [Lind- |
Ultrasound has several uses in medicine. The most com- |
|||||||||||||
say and Beyer (1989); Denny (1993)]. Sound that we can |
||||||||||||||
mon is to provide diagnostic images that complement |
||||||||||||||
hear (in the frequency range of 20 Hz to 20 kHz) is atten- |
||||||||||||||
those made with x-rays, nuclear medicine, and magnetic |
||||||||||||||
uated by about 0.1–10 dB km−1. Water transmits sound |
||||||||||||||
resonance. Another is to heat tissue (diathermy). It is |
||||||||||||||
better than air, but its attenuation is an even stronger |
||||||||||||||
also used to |
break up |
gall stones |
and |
kidney |
stones |
|||||||||
function of frequency. It also depends on the salt con- |
||||||||||||||
(lithotripsy), and, experimentally, to destroy tissue by in- |
||||||||||||||
tent. At 1, 000 Hz, sound attenuates in fresh water by |
||||||||||||||
tense heating. |
|
|
|
|
|
|
|
|
||||||
about 4 ×10−4 dB km−1. The attenuation in sea water is |
|
|
|
|
|
|
|
|
||||||
The |
highest-frequency sounds |
|
that |
we |
can |
hear |
||||||||
about a factor of ten higher [Lindsay and Beyer (1989)]. |
(≈15 kHz) have a wavelength in water of 0.1 m. One |
|||||||||||||
The low attenuation of sound in water (especially at low |
||||||||||||||
frequencies) allows aquatic animals to communicate over |
property of waves is that di raction limits our ability to |
|||||||||||||
produce an image. Only objects larger than or approxi- |
||||||||||||||
large distances [Denny (1993)]. |
|
|||||||||||||
|
mately equal to the wavelength can be imaged e ectively. |
|||||||||||||
The attenuation of sound depends strongly |
on fre- |
|||||||||||||
This property is what limits light microscopes (using elec- |
||||||||||||||
quency. Figure 13.11 shows some representative values. |
||||||||||||||
tromagnetic waves to form an image) to resolutions equal |
||||||||||||||
As a rule of thumb, at ultrasonic frequencies the atten- |
||||||||||||||
to about the wavelength of visible light, 500 nm. If we |
||||||||||||||
uation is proportional to frequency, with the constant |
||||||||||||||
used audible sound to form images, our resolution would |
||||||||||||||
of proportionality being 100 dB m−1 MHz−1. There are |
||||||||||||||
be limited to about 0.07 m, which would be a poor im- |
||||||||||||||
large variations in attenuation in tissue, depending on the |
||||||||||||||
age indeed. To overcome this di culty, higher frequencies |
||||||||||||||
age of the subject and other factors. Values can be found |
||||||||||||||
(ultrasound) are used to form images. Typically, diag- |
||||||||||||||
in Appendix A of ICRU 61 (1998). |
|
|||||||||||||
|
nostic ultrasound uses frequencies on the order of 1 to |
|||||||||||||
There can also be scattering of the sound from some |
||||||||||||||
15 MHz, corresponding to wavelengths of 1.4 to 0.1 mm |
||||||||||||||
object, just as there is for light. The total scattering cross |
||||||||||||||
in tissue. Higher frequencies would result in even shorter |
||||||||||||||
section for the object is defined by |
|
|||||||||||||
|
wavelengths, but higher frequency sound has increased |
|||||||||||||
|
|
|||||||||||||
Ws |
|
attenuation, which ultimately sets an upper bound to the |
||||||||||||
σs = I0 , |
(13.38) |
useful frequency. |
|
|
|
|
|
|
|
where Ws is the total power scattered and I0 is the incident intensity. As in Chapter 14, the di erential scattering cross section can also be defined.
5ICRU 61 (1998).
6Sometimes the attenuation coe cient is expressed in nepers m−1, in which case the natural logarithm of the intensity or pressure ratio is used.




354 13. Sound and Ultrasound
of sound is c. At t = 0, the source emits the crest of |
l |
Length |
m |
|
|
344 |
|||||||||
a wave with period T (frequency f = 1/T ). The wave |
p |
Excess pressure |
Pa |
|
344 |
||||||||||
travels to the right. This crest takes a time t = L/c to |
sn |
Normal stress |
Pa |
|
344 |
||||||||||
reach a stationary receiver a distance L away. At t = T , |
r, r |
Position |
m |
|
|
351 |
|||||||||
t |
Time |
s |
|
|
344 |
||||||||||
one period later, another crest is emitted by the source. |
|
|
|||||||||||||
v |
Fluid or particle |
m s−1 |
|
345 |
|||||||||||
This crest takes less time to reach the receiver because |
|
velocity |
|
|
|
|
|||||||||
the source has moved closer to the receiver. Specifically, |
|
|
|
|
|
||||||||||
vs, vo |
Velocity of |
m s−1 |
|
354 |
|||||||||||
the distance from source to receiver is now L − vsT , so |
|
source, observer |
|
|
|
|
|||||||||
the crest reaches the receiver at t = T + (L − vsT )/c. |
x, y, z |
Position |
m |
|
|
343 |
|||||||||
The time T between crests reaching the receiver is T = |
E |
Young’s modulus |
Pa |
|
344 |
||||||||||
T + (L − vsT )/c − L/c = T (1 − vs/c). The frequency |
F |
Force |
N |
|
2 |
344 |
|||||||||
|
|
|
W m− |
|
|||||||||||
observed by the receiver is |
|
|
|
|
I |
Intensity |
|
347 |
|||||||
|
|
|
|
|
|
|
|
J1 |
Bessel function of |
|
|
|
352 |
||
|
f = 1/T = |
|
f |
(13.41) |
|
order 1 |
|
|
|
|
|||||
|
|
|
|
. |
L |
Distance |
m |
|
|
354 |
|||||
|
|
|
|
|
|
||||||||||
|
1 |
− vs/c |
|
M |
Mass |
kg |
|
345 |
|||||||
If the source is moving toward the receiver with a speed |
P |
Pressure |
Pa |
|
344 |
||||||||||
R |
Reflection |
|
|
|
347 |
||||||||||
equal to 10% of the speed of sound, then f |
is about 11% |
|
|
|
|||||||||||
|
coe cient |
|
|
|
|
||||||||||
higher than f . When the source is moving away from the |
|
|
|
|
|
||||||||||
S |
Area |
m |
2 |
|
344 |
||||||||||
receiver, f |
= f /(1 + vs/c) (see Problem 32). It is not |
|
|
||||||||||||
T |
Transmission |
|
|
|
347 |
||||||||||
di cult to include the e ect of motion of the reflecting |
|
coe cient |
|
|
|
|
|||||||||
surface at an angle with the ultrasound beam. |
T |
Period |
s |
|
|
354 |
|||||||||
In medical ultrasound applications, the detected wave |
V |
Volume |
m3 |
|
344 |
||||||||||
is usually a reflection from moving tissue, such as red |
Ws |
Power scattered |
W |
|
350 |
||||||||||
blood cells. In this case, the relationship between the fre- |
Z |
Acoustic |
Pa s m−1or kg |
346 |
|||||||||||
quency f produced by a stationary source and the fre- |
|
impedance |
m−2s−1 |
|
|||||||||||
α |
Amplitude atten- |
m−1 |
|
350 |
|||||||||||
quency f received by the stationary receiver after reflec- |
|
||||||||||||||
|
uation coe cient |
|
|
|
|
||||||||||
tion from an object moving away from it at speed vo is |
|
|
|
|
|
||||||||||
κ |
Compressibililty |
Pa−1 |
|
344 |
|||||||||||
(see Problem 33) |
|
|
|
|
n |
Normal strain |
|
|
|
344 |
|||||
|
|
1 − vo/c |
|
|
|
λ |
Wavelength |
m |
|
|
345 |
||||
|
f = f |
. |
(13.42) |
µ |
Intensity attenua- |
m−1 |
|
350 |
|||||||
|
|
1 + vo/c |
|
|
tion coe cient |
kg m−3 |
|
||||||||
|
|
|
|
|
|
|
|
ρ |
Density |
344 |
|||||
The di erence in frequency between f and f contains |
σ |
Scattering cross |
m2 |
|
350 |
||||||||||
information about the speed of the object (Problem 35). |
|
section |
|
|
|
|
|||||||||
Doppler ultrasound is often used in medicine to measure |
θ |
Angle |
m |
|
|
352 |
|||||||||
speed, such as the speed of moving blood cells. Often the |
ξ |
Displacement |
|
|
344 |
||||||||||
|
from equilibrium |
|
|
|
|
||||||||||
Doppler shift is measured for a pulse of ultrasound, so |
|
|
|
|
|
||||||||||
ω |
Angular |
s−1 |
|
345 |
|||||||||||
that one can be sure of the depth at which the Doppler |
|
||||||||||||||
|
frequency |
|
|
|
|
||||||||||
shift occurred. A distribution of red cell velocities can |
|
|
|
|
|
||||||||||
|
|
|
|
|
|
|
|||||||||
be measured by looking at the Doppler shift frequency |
Problems |
|
|
|
|
||||||||||
spectrum. |
|
|
|
|
|
|
|
|
|
|
|
||||
Symbols Used in Chapter 13 |
|
Section 13.1 |
|
|
|
|
|||||||||
|
Problem 1 Show that 1/√ |
|
has units of speed. |
||||||||||||
|
|
|
|
|
|
|
|
ρ0κ |
|||||||
Symbol |
Use |
Units |
First |
Problem 2 Show that the pressure p satisfies the wave |
|||||||||||
|
|
|
|
|
|
|
used on |
equation. Hint: Use Eqs. 13.13 and 13.14. Di erentiate |
|||||||
a |
Transducer radius |
m |
page |
to obtain ∂2p/∂x2 and ∂2p/∂t2. Also use the fact that |
|||||||||||
351 |
when multiple partial derivatives are taken, the order of |
||||||||||||||
c |
Speed of sound |
m s−1 |
344 |
||||||||||||
di erentiation can be interchanged (Appendix N). |
|||||||||||||||
f, g |
Arbitrary |
|
|
|
345 |
||||||||||
|
|
|
|
|
|
|
|
|
|
||||||
|
functions |
|
|
|
|
Problem 3 Show that v and |
ρ also satisfy |
the wave |
|||||||
f, f |
Frequency |
Hz |
345 |
||||||||||||
h |
Arbitrary |
|
|
|
345 |
equation. |
|
|
|
|
|
|
|||
|
function |
|
|
|
|
|
|
|
|
|
|
|
|||
k |
Wave number |
m−1 |
345 |
Problem 4 Derive Eq. 13.15. |
|
|
|
|
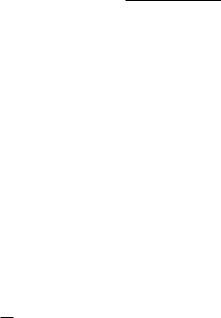