
Neutron Scattering in Biology - Fitter Gutberlet and Katsaras
.pdf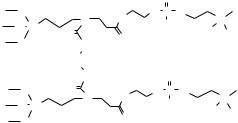
13 Protein Adsorption and Interactions at Interfaces |
275 |
a substantial structural deformation. The structural deformation is consistent with the large extent of irreversible adsorption observed. These structural features may lead to clues as to why traditional hydroxy-based methacrylate polymers are not the best candidates as biomaterials for contact lenses and implant coatings.
In the course of studying protein adsorption on SAMs bearing usual terminal chemical functions, we also developed synthetic schemes for chemical attachment of SAMs bearing terminal phosphorylcholine (PC) groups. Adsorption at PC monolayer–solution interface [31] was pursued in parallel to the PC polymer films [32–34] carried out in collaboration with Biocompatibles UK Ltd. A particular version of PC molecules used for surface chemical grafting onto SiO2 surface is shown in Fig. 13.8. The coated surface gave a typical advancing contact of some 30◦ and was found to be very e ective at reducing protein adsorption. These SAM surfaces were found to be as e ective as ultrathin PC polymer films at reducing protein adsorption. However, when compared with other more conventional polymeric materials, the reduction of nonspecific protein deposition by PC materials is more substantial. While these features can be attributed to the chemical nature of di erent materials, they set challenges for us to seek more appropriate explanations. It is relevant to note that while both PC and C15OH surfaces reduce protein adsorption substantially, the structural conformations of the adsorbed protein layers were di erent. The C15OH–water interface induces strong deformation of lysozyme, but this is in contrast to the loose lysozyme distribution over 80–100 ˚A at the PC monolayer–water interface, indicating reduced contacts between the protein molecules and the PC surface. This feature is thought to arise from the high-surface hydration of PC groups, creating a hydration barrier. This structural characteristic is in agreement with the high degree of reversibility of adsorption on this surface.
Adsorption of other proteins such as BSA, HSA, and IgG were found to show similar behavior to lysozyme, e.g., the attainment of maximal adsorption at the solution pH close to their IPs [35, 36]. However, as the size goes up and stability goes down, the influence of pH becomes less obvious. It was also
|
|
|
O |
|
O |
N |
O |
O P O |
+ |
O Si |
− |
N |
||
O |
|
O |
|
|
O |
O |
|
|
|
NH |
|
|
||
|
|
|
||
|
|
|
|
|
|
(H2C)6 |
|
|
|
|
NH |
|
O |
|
O |
O |
O |
O P O |
+ |
O Si |
N |
− |
N |
|
|
|
O |
|
OO
PC Dimer
Fig. 13.8. Molecular structure of a PC dimer used for surface grafting onto SiO2
276 J.R. Lu
observed that upon adsorption onto PC monolayer surface, the greater the protein size, the further reduced the adsorption. This trend is opposite when proteins are adsorbed on surfaces such as OTS and SiO2. However, the exact nature of this behavior remains unclear. As the size increases, the extent of reversibility decreases, as expected. The experimental methodology developed here will add to the concerted e ect in the understanding of the molecular mechanistic processes underlying surface biocompatibility [37–42].
Exchange of labile hydrogens in protein molecules with bulk D2O is an issue that deserves some proper discussion. Uncertainty in the extent of incomplete exchanges would a ect the surface excess although this has no e ect on layer thickness. When proteins retain their globular structures, some labile hydrogens on the amino acid side chains and the backbones may be inhibited to exchange because of hydrogen bonding and hydrophobic encapsulation [43]. We have shown that for both lysozyme and BSA, the exchanges with bulk D2O are complete within a few percent. We have further supported this verdict by comparing the protein surface excesses before and after structural unfolding induced by sodium dodecyl sulphate (SDS) [44,45]. Since H/D exchanges have been widely used as a probe for detecting structural perturbations, this experiment shows that neutron reflection has the potential for following the H/D exchanges with time when this e ect is su ciently slow (in the timescale of 10 min and beyond).
In summary, the main advance we have made in this part of study is the demonstration of simultaneous determination of in situ protein layer structure and composition at the solid–solution interface. This information together with the known three-dimensional structures of proteins allows reliable assessment of the extent of protein deformation and unfolding to be made. Although the deuteration of proteins is di cult, we have shown that by appropriate use of solvent isotopic contrasts, di erent parts of the interfacial layer can be highlighted and their structural distributions measured with su cient resolution. We can summarize the main observations as follows:
–Protein molecules retain their globular frameworks at the hydrophilic solid–water interface but unfold completely at the hydrophobic solid–water interface. The amount of adsorption on these interfaces may however be comparable.
–Surfaces with intermediate hydrophobicity show substantially reduced adsorption, but the structural conformations of the adsorbed protein layers are di erent between the hydroxy (−C15H30OH) and phosphorylcholine (PC)-terminated surfaces, indicating the subtle e ects of the nature of surface chemistry.
–Adsorption tends to reach maximum around the isoelectric point (IP) of the protein.
–The extent of structural deformation and degree of irreversibility of adsorption increases with the size of proteins.
–Labile hydrogens within globular proteins are completely exchanged when adsorbed at the interfaces.
13 Protein Adsorption and Interactions at Interfaces |
277 |
13.5 Interaction Between Surfactant and Protein
In this section first the coadsorption of protein and surfactant at the air– water interface will be introduced, again because this surface can be used as model to examine the nature of the interaction without the interfering effect from the solid surface. An extensive work carried out previously [2] has indicated the e ect of surfactant head groups on the mode of di ering interactions. The representative data from the coadsorption of nonionic surfactant is shown. Although our previous work has shown that the binary mixture of lysozyme and nonionic C12E5 produced a typical model of competitive adsorption, structural deformation of lysozyme was revealed during the coadsorption at the surface [46]. The structural detail concerning lysozyme deformation was probed using hydrogenated C12E5 adsorbed from NRW. Under this isotopic contrast the reflectivity obtained arose from the lysozyme layer with little contribution from the hydrogenated C12E5.
When anionic SDS was used, completely di erent interfacial processes were observed [47]. Surface tension measurements indicated a rather complicated interfacial event. Just relying on surface tension alone, it would be impossible to unravel the key interfacial molecular processes and be able to outline the main picture. The surface tension shown in Fig. 13.9a is marked by a maximum around 1 mM SDS, followed by a break point well below the CMC of pure SDS. It is useful to comment that in the absence of the neutron data the apparent discontinuity at the low SDS concentration would be attributed to the onset of formation of micelles on the protein. The neutron reflection measurement, however, shows that over this region there is a steady increase of adsorbed amount of both lysozyme shown in Fig. 13.9b and SDS shown in Fig. 13.9c, with increasing SDS concentration. Thus the discontinuity is associated with the formation of highly surfaceactive SDS–lysozyme complexes and has nothing to do with the micellization on the protein. The almost constant surface tension over the SDS concentration between 0.05 and 0.5 mM conceals a more complex surface behavior, which is clearly indicated by the dramatic variation of the adsorbed amount of both SDS and lysozyme (Fig. 13.9b, c), as revealed by neutron reflection. These results together show that electrostatic interactions determine the low-surfactant concentration behavior and hydrophobic interactions prevail over the high surfactant concentration range. The combination of interactions over the crossover region can give rise to a range of quite di erent e ects, including multilayer formation. The neutron data is able to reveal the role of the electrostatic interactions very clearly, not just by monitoring the composition of the surface but also by leading to the complex structure of the composite layer. The density profiles for the protein itself over the lowest SDS concentration region are approximately uniform and comparable with lysozyme on its own, indicating that no major structural deformation occurs. Thus, it appears that, in this case, the strong electrostatic interaction has a large e ect on the thermodynamic behavior but is not strong enough to induce loss of tertiary structure. Further SDS
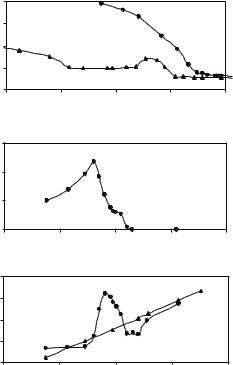
278 |
J.R. Lu |
(a) |
N/m) |
70 |
|
|
|
|
|
|
|
|
|
||
|
|
|
|
|
|
|
|
(nm |
60 |
|
|
|
|
|
|
|
|
|
|
|
|
tension |
50 |
|
|
|
|
|
40 |
|
|
|
|
|
|
Surface |
|
|
|
|
|
|
30 |
−10 |
−8 |
−6 |
−4 |
|
|
−12 |
|||||
|
|
|
|
|
|
Ln (SDS/M)
(b) |
|
Lysozymesurfaceexcess |
(mg/m2) |
6
4
2
0−12 |
−10 |
−8 |
−6 |
−4 |
|
|
Ln (SDS/M) |
|
|
(c) excesssurface
SDS
(mg/m2)
1,6 |
|
|
|
|
1,2 |
|
|
|
|
0,8 |
|
|
|
|
0,4 |
|
|
|
|
0 |
−10 |
−8 |
−6 |
−4 |
−12 |
Ln (SDS/M)
Fig. 13.9. (a) Surface tension for pure SDS (•) and SDS–lysozyme mixture ( ), (b) surface excess for lysozyme and (c) surface excess of SDS measured on the surface of binary mixture (•) as compared with its excess in pure form ( )
addition into the “hydrophobic” region leads to the breakdown of the lysozyme framework as the hydrophobic interactions build up enough to dominate the electrostatic interactions.
Interactions at the solid–solution interface have mainly been done using the hydrophilic SiO2, using preadsorbed protein layers in contact with pure surfactant solution. This experimental process mimics the cleaning of medical devices well. The results show that while nonionic surfactant such as C12E5 shows little tendency of association with preadsorbed proteins, both anionic and cationic surfactants interact strongly, resulting in di erent extent of protein removal. The high sensitivity of neutron reflectivity is well demonstrated from SDS binding to preadsorbed BSA, and this study was performed in conjunction with SDS labeling [44,45]. The modeling of reflectivities clearly shows that the interfacial mixtures are unevenly distributed, with SDS distributions skewed towards the bulk water. The studies also show that the removal of the adsorbed protein does not start until a critical SDS concentration is reached,
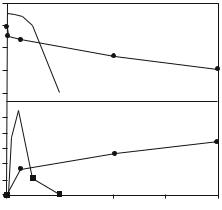
13 Protein Adsorption and Interactions at Interfaces |
279 |
that the critical concentration varies with pH and salt concentration and that binding of SDS induces structural unfolding of the preadsorbed protein.
SDS binding to the immobilized lysozyme was also studied to compare the e ect of the nature of protein [48]. A significant di erence was observed between the extent of surfactant bound to BSA and lysozyme. For SDS–BSA system, the amount of SDS bound to each gram of protein adsorbed at the interface (weight ratio) was 0.43, close to that found by Tanford et al. [49] for the binding carried out under similar bulk solution conditions. This is in contrast to the observed weight ratio of 0.1 for SDS–lysozyme. This di erence is clearly attributable to the nature of the proteins, although Tanford et al. have shown that in bulk solution these di erences, if any, are much smaller. These results together enforce the view that the interactions at the interface are very di erent from bulk solution. This statement is consistent with the fact that the surfactant–protein interaction is nonideal and that the interfacial structure and composition is not expected to be the same as in bulk solution.
Figure 13.10 depicts the pattern of protein removal from the hydrophilic SiO2 surface using anionic SDS and cationic C12TAB (dodecyltrimethyl ammonium bromide) [50]. Clearly, when C12TAB is used, it can progressively remove lysozyme, but the extent of removal never reaches completion. Also, with the increasing lysozyme removal, the interfacial excess of C12TAB tends to increase, indicating the preferential binding of C12TAB to the interface. The results shown in Fig. 13.10 clearly show the e ect of surfactant head groups and the underlying di erences in the molecular processes of interfacial interactions.
These examples and other related preliminary work show that neutron reflection can provide better explanations of the molecular processes involved
|
4 |
|
|
|
|
|
|
|
|
Lysozyme surface |
3 |
|
|
|
|
|
|
|
(a) |
|
|
|
|
|
|
|
|||
|
|
|
|
|
|
|
|
||
|
|
|
|
|
|
|
|
|
|
excess (mg/m3) |
2 |
|
|
|
|
|
|
|
|
|
1 |
|
|
|
|
|
|
|
|
|
0 |
|
|
|
|
|
|
|
|
|
|
|
|||||||
|
|
|
|||||||
Surfactant surface |
0.5 |
|
|
|
|
|
|
|
(b) |
|
|
|
|
|
|
|
|||
|
|
|
|
|
|
|
|||
excess (mg/m3) |
0.4 |
|
|
|
|
|
|
|
|
|
|
|
|
|
|
|
|
||
|
|
|
|
|
|
|
|
|
0.3 |
|
|
|
|
0.2 |
|
|
|
|
0.1 |
|
|
|
|
0 |
|
|
|
|
0 |
1/4 |
1/2 |
3/4 |
1 |
|
|
Surfactant (cmc) |
|
|
Fig. 13.10. Comparison of protein elution capabilities of SDS ( ) and C12TAB (•) shown as the variation of surface excess of lysozyme (a) and surfactant (b) with bulk surfactant concentration
280 |
J.R. Lu |
in protein–surfactant complexation. The complexity of the interactions both at the surface and in bulk solution is such that no reliable model can be established in the absence of a correct description of the complexation at the interfaces. This statement lends its support from further neutron reflection studies of surfactant complexation with protein at the hydrophobed solid– solution interface where the pattern of interactions is very di erent from the data obtained at the hydrophilic SiO2–solution interface.
13.6 Future Prospects
Although extensive studies have been made on the interactions between proteins and surfactants in bulk solution, less is known about their behavior at the interfaces. It is important to realize that the nonideal behavior of protein adsorption and protein–surfactant interactions at the interfaces cannot be predicted by understanding their behavior from bulk solution because of interfacial e ects and more importantly, because of our inability in predicting how and when a protein molecule deforms and unfolds when it arrives at a given interface. The current work provides a useful experimental methodology for further research into the understanding of molecular mechanistic processes related to surface-induced structural deformation and denaturation. The strong relevance of this research to a wide range of conventional and emerging technological applications will stimulate computational e ort and theory development to attempt to corroborate the structural information obtained in neutron reflection with macroscopic behavior.
Acknowledgements
The author would like to thank the financial support from Biocompatibles UK Ltd, BBSRC, EPSRC. Thanks also go to his past and present research students, collaborators, mentors for their assistance, support, and direction. We thank the American Chemical Society (ACS) for the permission to use Figs. 13.5, 13.6, 13.9, and 13.10.
References
1.M. Malmsten, Biopolymers at Interfaces, 2nd Ed, Revised and Expanded, Surf. Sci. Ser. 110 (Dekker, New York, 2003)
2.T.A. Horbett, J.L. Brash, Proteins at Interfaces II, ACS Symposium Series 602 (Am. Chem. Soc., Washington DC, 1995)
3.K.B. McClary, T. Ugarova, D.W. Grainger, J. Biomed. Mater. Res. 50 (2000), 428
4.J. Andrade, Surface and Interfacial Aspects of Biomedical Polymers, Vol. 2 (Plenum, New York, 1985)
13 Protein Adsorption and Interactions at Interfaces |
281 |
5.R.J. Green, R.A. Frazier, K.M. Shakeshe , M.C. Davies, C.J. Roberts, S.J.B. Tendler, Biomaterials 21 (2000), 1823
6.E. Ostuni, B.A. Grzybowski, M. Mrksich, C.S. Roberts, G.M. Whitesides, Langmuir 19 (2003), 1861
7.J.L. Keddie, Curr. Opin. Colloid Interface Sci. 6 (2001), 102
8.R.J. Green, I. Hopkinson I, R.A.L. Jones, Langmuir 15 (1999), 5102
9.J. Kim, G.A. Somorjai, J. Am. Chem. Soc. 125 (2003), 3150
10.T.J. Su, J.R. Lu, Z.F. Cui, R.K. Thomas, J. Membr. Sci. 173 (2000), 167
11.J.C. Marshall, T. Cosgrove, K. Jack, A. Howe, Langmuir 18 (2002), 9668
12.A. Kondo, F. Murakami, K. Higashitani, Biotech. Bioeng. 40 (1992), 889
13.M.H. Tian, W.K. Lee, M.K. Bothwell, J. McGuire, J. Colloid Interface Sci. 200 (1998), 146
14.P. Billsten, P.O. Freskgard, U. Carlsson, B.H. Jonsson, H. Elwing, FEBS Lett. 402 (1997), 67
15.J.R. Lu, E.M. Lee, R.K. Thomas, Acta Cryst. A52 (1996), 11
16.J.R. Lu, R.K. Thomas, J. Chem. Soc., Faraday Trans. 94 (1998), 995
17.J.R. Lu, R.K. Thomas, in Physical Chemistry of Biological Interfaces, Eds. A. Baszkin, W. Norde, (Dekker, New York, 2000) p. 609–650
18.J.R. Lu, Annu. Rep. Prog. Chem. C 95 (1999), 3
19.J.R. Lu, S. Perumal, E. Powers, J. Kelly, J. Webster, J. Penfold, J. Am. Chem. Soc. 125 (2003), 3751
20.J.R. Lu, T.J. Su, R.K. Thomas, J. Penfold, J. Webster, J. Chem. Soc., Faraday Trans. 94 (1998), 3279
21.J.R. Lu, T.J. Su, B. Howlin, J. Phys. Chem. B 103 (1999), 5903
22.J.R. Lu, T.J. Su, J. Penfold, Langmuir 15 (1999), 6975
23.J.R. Lu, T.J. Su, R.K. Thomas, J. Colloid Interface Sci. 213 (1999), 426
24.J.B. Brzoska, N. Shahidzadeh, F. Rondelez, Nature 360 (1992), 719
25.T.J. Su, J.R. Lu, R.K. Thomas, Z.F. Cui, J. Penfold, Langmuir 14 (1998), 438
26.T.J. Su, J.R. Lu, R.K. Thomas, Z.F. Cui, J. Penfold, J. Colloid Interface Sci. 203 (1998), 419
27.C.A. Haynes, E. Sliwinski, W. Norde, J. Colloid Interface Sci. 164 (1994), 394
28.C.A. Haynes, W. Norde, J. Colloid Interface Sci. 169 (1995), 313
29.J.R. Lu, T.J. Su, R.K. Thomas, A.R. Rennie, R. Cubit, J. Colloid Interface Sci. 206 (1998), 212
30.T.J. Su, R.J. Green, Y. Wang, E.F. Murphy, J.R. Lu, R. Ivkov, S.K. Satija, Langmuir 16 (2000), 4999
31.J.R. Lu, E.F. Murphy, T.J. Su, A.L. Lewis, P.W. Stratford, S.K. Satija, Langmuir 17 (2001), 3382
32.E.F. Murphy, J.L. Keddie, J.R. Lu, J. Brewer, J. Russell, Biomaterials 20 (1999), 1501
33.E.F. Murphy, J.R. Lu, A.L. Lewis, J. Brewer, J. Russell, P. Stratford, Macromolecules 33 (2000), 4545
34.E.F. Murphy, J.R. Lu, J. Brewer, J. Russell, J. Penfold, Langmuir 15 (1999), 1313
35.T.J. Su, J.R. Lu, R.K. Thomas, Z.F. Cui, J. Phys. Chem. B 103 (1999), 3727
36.T.J. Su, J.R. Lu, R.K. Thomas, Z.F. Cui, J. Penfold, J. Phys. Chem. B 102 (1998), 8100
37.K.L. Prime, G.M. Whitesides, Science 252 (1994), 1164
38.C.M. Roth, A.M. Lenho , Langmuir 11 (1995), 3500
282J.R. Lu
39.K.L. Prime, G.M. Whitesides, J. Am. Chem. Soc. 115 (1993), 10714
40.S. Herrwerth, W. Eck, S. Reinhardt, M. Grunze, J. Am. Soc. Chem. 125 (2003), 9359
41.Y. Iwasaki, A. Fujike, K. Kurita, K. Ishihara, N. Nakabayashi, J. Biomater. Sci. Polym. Edn. 8 (1996), 91
42.A.L. Lewis, Colloid Surf. B, Biointerfaces 18 (2000), 261
43.S.E. Radford, M. Buck, K.D. Topping, C.M. Dobson, P.A. Evans, Proteins: Struct. Func. Gene. 14 (1992), 237
44.J.R. Lu, T.J. Su, R.K. Thomas, J. Phys. Chem. B 102 (1998), 10307
45.J.R. Lu, T.J. Su, R.K. Thomas, J. Penfold, Langmuir 14 (1998), 6261
46.R.J. Green, T.J. Su, J.R. Lu, J. Webster, J. Penfold, Phys. Chem. Chem. Phys. 2 (2000), 5222
47.R.J. Green, T.J. Su, H. Joy, J.R. Lu, Langmuir 16 (2000), 5797
48.R.J. Green, T.J. Su, J.R. Lu, J. Penfold, J. Phys. Chem. B 105 (2001), 1594
49.C. Tanford, J. Mol. Biol. 67 (1972), 59
50.R.J. Green, T.J. Su, J.R. Lu, J.R.P. Webster, J. Phys. Chem. B 105 (2001), 9331

14
Complex Biomimetic Structures
at Fluid Surfaces
and Solid–Liquid Interfaces
T. Gutberlet, M. L¨osche
14.1 Introduction
One major contributor to the recent success of nanotechnology is its profoundly interdisciplinary nature, involving supramolecular chemistry, biotechnology, bioinspired materials science, large-scale computational methods, and physical characterization techniques. One important role of physics in this context is the development of characterization methods that are su ciently sensitive for the investigation of ever smaller sample sizes – down to monomolecular sensitivity – and ever smaller sample dimensions, as well as techniques that probe directly the relevant intermolecular and intramolecular interactions. A variety of surface and interface sensitive techniques, such as scanning force and fluorescence microscopy, FT-IRRAS, XPS, surface plasmon spectroscopy and surface-sensitive X-ray and neutron scattering have been developed in the past 15 years to reveal such information on nanoscopic systems. Planar lipid membrane mimics, such as floating monolayers on aqueous surfaces or immobilized bilayers on solid supports, have been established to correlate structural, functional and dynamic aspects of biomembrane models [1].
Neutron and X-ray scattering in nanotechnology share common grounds. The two techniques closely related to each other not only in terms of the underlying physics – neutrons scatter from the nuclei of a molecular species while X-rays scatter from their electron clouds – but also the formalisms that quantitate the optics of these processes are very similar. Neutrons and X- rays are also largely synergistic in their application to the characterization of interfacial structures [2,3]. Neutrons feature distinctive advantages over X-rays due to the possibility of isomorphic contrast variation and their penetration into condensed matter for the characterization of deeply buried interfaces [4,5]. Following the advent of third-generation synchrotron sources [6], however, in particular X-ray techniques have been an indispensable tool that has driven the development of nanoscience. Current perspectives of significant increases in neutron flux at sources under construction or in the planning stage [7,8] on the other hand, is expected to boost the application of neutrons in nanoscience
284 T. Gutberlet et al.
considerably and will thus increase the overall capabilities of surface-sensitive scattering even further.
In this chapter, we review recent achievements in the molecular-level characterization of bioinspired interface and surface architectures using surfacesensitive neutron scattering and will briefly describe practical aspects of the application of reflectivity techniques. Then we will survey recent work with the aim of exemplifying the capabilities of neutrons in the elucidation of submolecular structures. For a deeper discussion of experimental and technical aspects of neutron scattering at interfaces, we refer to the contribution by Majkrzak and coauthors in this volume. For a review on aspects of protein adsorption and their interactions at interfaces, the reader is referred to the contribution by Lu, in this volume.
14.2 Surface-Sensitive Scattering
As reviewed elsewhere [9], surface-sensitivity in a scattering experiment, i.e., the discrimination of scattering from an interface-associated layer of (organic) material of nanometer thickness against the vast background of molecules contained in the adjacent bulk phase, is generally achieved by impinging the beam at grazing angles. Near the critical angle for total external reflection, this creates an evanescent wave which penetrates the bulk phase only by a few 10 nm [10]. This enables three classes of experiments: (i) specular and (ii) o -specular reflection, as well as (iii) grazing-incidence (“in-plane”) diffraction (GID) (Fig. 14.1). Of these generic interface-sensitive scattering techniques, only specular reflection plays a role in the neutron scattering from organic monolayers, as the cross-sections for nonspecular scattering and for GID are orders of magnitude lower than that for specular scattering. The latter experiments are thus currently limited to synchrotron X-ray experiments (grazing-incidence X-ray di raction GIXD). Specular reflection of neutrons at interfaces, however, is developing into a work horse in biologically oriented nanoscience.
14.2.1 Specular Reflectivity
As discussed by Majkrzak et al., in this volume, specular reflectivity reveals only one-dimensional (1D) information on the scattering length density (SLD) at an interface. Another limitation is that the method does not provide atomic- scale information on the system, comparable to, e.g., X-ray crystallography. Advanced structure-based data analysis techniques, however, provide a quasi2D structural assessment of the investigated system, as delineated below. In addition, such techniques provide a framework for the consistent mapping of di erent contrasts onto each other. Thus, the simultaneous evaluation of neutron data taken from samples with di erent deuteration patterns or of neutron and X-ray data sets from identically prepared systems enhance greatly