
Neutron Scattering in Biology - Fitter Gutberlet and Katsaras
.pdf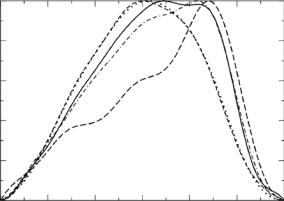
182 S. Krueger et al.
1
0.8
0.6
P (r)
0.4
0.2
0
0 |
50 |
100 |
150 |
200 |
250 |
300 |
|
|
|
r (Å) |
|
|
|
Fig. 9.13. Distance distribution functions, P (r) vs. r, from the MS2 data for samples in 100% (solid line), 85% (dot-dashed line), 65% (long dashed line), 10% (dashed line), and 0% D2O (dotted line)
P (r) functions suggest that the particle does actually extend beyond 2 × R2. However, the number of probable distances beyond 2 × R2 drops sharply.
The P (r) function for the 65% D2O sample is consistent with that of a hollow spherical shell. In this case, the peak of the distance distribution is at 200 ˚A, consistent with the fact that the most probable distances are occurring beyond 2 × R1. On the other hand, the peak of the distance distribution function occurs at values smaller than 2×R1 for the data obtained at the other contrasts. The RNA component is contributing more to the total scattering in 0% and 10% D2O and this is reflected as a shift in the peak in P (r) to smaller r values. In 85% and 100% D2O, the RNA component is contributing to the total scattering, but the scattering from the protein component is much stronger. Thus, the peak in P (r) falls in between the 65% D2O case and the 0% and 10% D2O cases.
The scattered intensities from the RNA and protein components were separated from each other using Eq. 9.3. The resultant P (r) functions are shown in Fig. 9.14. While Dmax for the protein shell remains at 300 ˚A, Dmax for the RNA core was found to be 165 ˚A. Thus, the RNA component appears to be confined mostly within a radius of ≈83 ˚A. The peak of the RNA P (r) distribution is also around this value. These results agree very well with the R1 values from the core-shell model fits for the samples measured in 10% D2O. Recall, that the 10% D2O solvent condition is where the RNA scattering is the strongest relative to that of the protein. Indirect genetic and biochemical results hint that a variety of mechanisms may act in concert to fold and compact the MS2 genomic RNA. This body of in vitro experiments suggests strongly
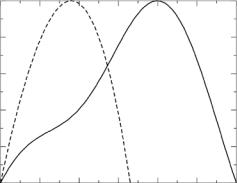
9 SANS from Proteins, Nucleic Acids, and Viruses |
183 |
1.0
0.8
0.6
P (r)
0.4
0.2
0.0
0.0 50.0 100.0 150.0 200.0 250.0 300.0 r (Å)
Fig. 9.14. Distance distribution functions, P (r) vs. r, for the protein (solid line) and RNA (dashed line) components of the MS2 particles
that the MS2 RNA is tightly compacted and that the degree of packing is important for transcriptional regulation and genomic integrity. This work is the first study to directly measure the spatial distribution of the MS2 genomic RNA under indigenous conditions and to confirm that it is indeed compact in vivo.
Acknowledgments
The work presented in this chapter is the result of close collaborations and we would like to take this opportunity to explicitly thank our collaborators. In particular, UPS and SK would like to thank Prof. Sarah Woodson, Dr. Pranshanth Rangan, Mr. Robert Moss, Prof. Deverajan Thirumalai and Prof. Robert Briber for the group e ort on the RNA work. SKG and SK would like to thank Prof. Edward Eisenstein and Mr. James Zondlo for their kind collaboration on the chaperonin project and Ms. Jing Zhou for her work in developing the LORES program. DK and SK would like to thank Drs. Charles Wick and Ilya Elashvili for collaborating with them on the MS2 bacteriophage work. Partial support for the RNA compaction research came from grants from the National Institutes of Health. UPS was supported by the National Research Council/National Institute of Standards and Technology Postdoctoral Associateship Program. SKG thanks the DOE/Sloan Foundation for a postdoctoral fellowship in computational molecular biology that helped support her work. DK was supported by the National Research Council/National Institute of Standards and Technology Postdoctoral Associateship Program. The NIST SANS facilities are partially supported by the National Science Foundation under Agreement Nos. DMR-9423101 and DMR-9986442.
184 S. Krueger et al.
References
1.P.A. Timmins, G. Zaccai, Eur. Biophys. J. 15, 257–268 (1988)
2.J. Trewhella, Cur. Opin. Struct. Biol. 7, 702–708 (1997)
3.S. Krueger, Physica B 241, (1998) 1131–1137
4.A. Guinier, G. Fournet, Small-Angle Scattering of X-rays (John Wiley and Sons, 1955)
5.P.B. Moore, J. Appl. Cryst. 13, 168–175 (1980)
6.A.V. Semenyuk, D.I. Svergun, J. Appl. Crystallogr. 24, 537–540 (1991)
7.O. Glatter, J. Appl. Cryst. 10, 415–421 (1977)
8.D.I. Svergun, S. Richard, M.H.J. Koch, Z. Sayers, S. Kuprin, G. Zaccai, Proc. Natl Acad. Sci. USA 95, 2267–2272 (1998)
9.S. Hansen, J. Appl. Cryst. 3, 334–346 (1990)
10.D.B. Heidorn, J. Trewhella, Biochemistry 27, 909–915 (1988)
11.B. Jacrot, Rep. Prog. Phys. 39, 911–953 (1976)
12.S. Krueger, S. Gregurick, Y. Shi, S. Wang, B.D. Wladowski, F.P. Schwarz, Biochemistry 42, 1958–1968 (2003)
13.S.J. Perkins, Eur. J. Biochem. 157, 169–180 (1986)
14.J. Zhou, private communication
15.http://www.sans.chem.umbc.edu/
16.H.B. Stuhrmann, A. Miller, J. Appl. Cryst. 11, 325–345 (1978)
17.C.J. Glinka, J.G. Barker, B. Hammouda, S. Krueger, J.J. Moyer, W.J. Orts, J. Appl. Cryst. 31, 430–445 (1998)
18.N.E. Gabriel, M.F. Roberts, Biochemistry 23, 4011–4015 (1984)
19.P. Schurtenberger, N. Mazer, W. Koenzig, J. Phys. Chem. 89, 1042–1049 (1985)
20.B. Carion-Taravella, J. Chopineau, M. Ollivon, S. Lesieur, Langmuir 14, 3767– 3777 (1998)
21.M. Johnsson, K. Edwards, Langmuir, 16, 8632–8642 (2000)
22.M. Ollivon, S. Lesieur, C. Grabrielle-Madelmont, M. Paternotre, Biochim. Biophys. Acta 1508, 34–50 (2000)
23.C.R. Sanders II, J.P. Schwonek, Biochemistry, 31, 8898–8905 (1992)
24.C.R. Sanders II, B.J. Hare, K.P. Howard, J.H. Prestegard, Prog. NMR Spectro. 26, 421–444 (1994)
25.C.R. Sanders II, J.H. Prestegard, Biophys. J. 58, 447–460 (1990)
26.J. Chung, J.H. Prestegard, J. Phys. Chem. 97, 9837–9843 (1993)
27.M.-P. Nieh, C.J. Glinka, S. Krueger, R.S. Prosser, J. Katsaras, Langmuir 17, 2629–2638 (2001)
28.M.-P. Nieh, C.J. Glinka, S. Krueger, R.S. Prosser, J. Katsaras, Biophys. J. 82, 2487–2498 (2002)
29.G. Gregoriadis, Trends Biotechol. 13, 527–537 (1995)
30.M.-P. Nieh, T.A. Harroun, V.A. Rughunathan, C.J. Glinka, J. Katsaras, Phys. Rev. Lett. 91, 158105 (2003)
31.J.B. Hayter, J. Penfold, Mol. Phys. 42, 109–118 (1981)
32.P. Brion, E. Westhof, Ann. Rev. Biophys. Biomol. Struct. 26, 113–137 (1997)
33.D. Thirumalai, N. Lee, S.A. Woodson, D.K. Klimov, Ann. Rev. Phys. Chem. 52, 751–762 (2001)
34.T.R. Sosnick, T. Pan, Curr. Opin. Struct. Biol. 13, 309–316 (2003)
35.V.K. Misra, D.E. Draper, Biopolymers 48, 113–135 (1998)
36.K.L. Buchmueller, A.E. Webb, D.A. Richardson, K.M. Weeks, Nat. Struct. Biol. 7, 362–366 (2000)

9 SANS from Proteins, Nucleic Acids, and Viruses |
185 |
37.S.L. Heilman-Miller, D. Thirumalai, S.A. Woodson, J. Mol. Biol. 306, 1157– 1166 (2001)
38.R. Russell, I.S. Millett, S. Doniach, D. Herschlag, Nat. Struct. Biol. 7, 367–370 (2000)
39.X. Fang, K. Littrell, X.-J. Yang, S.J. Henderson, S. Siefert, P. Thiyagarajan,
T.Pan, T.R. Sosnick, Biochemistry 39, 11107–11113 (2000)
40.R. Russell, I.S. Millett, M.W. Tate, L.W. Kwok, B. Nakatani, S.M. Gruner, S.G. Mochrie, V. Pande, S. Doniach, D. Herschlag, L. Pollack, Proc. Natl. Acad. Sci. USA, 99, 4266–4271 (2002)
41.P. Rangan, B. Masquida, E. Westhof, S.A. Woodson, Proc. Natl. Acad. Sci. USA, 100, 11574–1579 (2003)
42.P. Rangan, S.A. Woodson, J. Mol. Biol. 329, 229–238 (2003)
43.U.A. Perez-Salas, P. Rangan, S. Krueger, R.M. Briber, D. Thirumalai, S.A. Woodson, Biochemistry 46, 1746–1753 (2004)
44.V.K. Misra, D.E. Draper, J. Mol. Biol. 294, 1135–1147 (1999)
45.V.K. Misra, D.E. Draper, J. Mol. Biol. 317, 507–521 (2002)
46.D. Thirumalai, B.Y. Ha, Phys. Rev. A 46, R3012–R3015 (1992)
47.Z. Lin, E. Eisenstein, Proc. Natl. Acad. Sci. USA, 93, 1977–1981 (1996)
48.N.F. McLennan, A.S. Girshovich, N.M. Lissin, Y. Charters, M. Masters, Mol. Microbiol. 7, 49–58 (1993)
49.P. Thiyagarajan, S.J. Henderson, A. Joachimiak, Structure 4, 79–88 (1996)
50.R. Stegmann, E. Manakova, M. Rossle, H. Heumann, S.E. Nieba-Axmann, A. Pluckthun, T. Hermann, R.P. May, A. Wiedenmann, J. Struct. Biol. 121, 30–40 (1998)
51.K. Braig, Z. Otwinowski, R. Hegde, D.C. Boisvert, A. Joachimiak, A.L. Horwich, P.B. Sigler, Nature 371, 578–586 (1994)
52.I.S. Weissman, Chem. Biol. 2, 255–260 (1995)
53.S.G. Burston, J.S. Weissman, G.W. Farr, W.A. Fenton, A.L. Horwich, Nature 383, 96–99 (1996)
54.S. Krueger, S.K. Gregurick, J. Zondlo, E. Eisenstein, J. Struct. Biol., 141, 240–258 (2003)
55.J.F. Hunt, A.J. Weaver, S.J. Landry, L. Gierasch, J. Deisenhofer, Nature 379, 37–45 (1996)
56.P.G. Stockley, N.J. Stonehouse, K. Valegard, Int. J. Biochemistry 26, 1249– 1260 (1994)
57.B.L. Pasloske, C.R. Walkerpeach, R.D. Obermoeller, M. Winkler, D.B. DuBois,
J.Clinical Microbiol. 36, 3590–3594 (1998)
58.P.G. Stockley, R.A. Mastico, Methods Enzymol. 326, 551–569 (2000)
59.http://www.ambiondiagnostics.com/products/armored rna.html/
60.D. Eisenberg, Physical Chemistry with Applications to the Life Sciences (Benjamin/Cummings, 1979)
61.J. Sambrook, D.W. Russell, Molecular Cloning a Laboratory Manual (Cold Spring Harbor Press, 2001)
62.J.H. Strauss, R.L. Sinsheimer, J. Mol. Biol. 7, 43–54 (1963)
63.D.A. Kuzmanovic, I. Elashvili, C. Wick, C. O’Connell, S. Krueger, Structure 11, 1339–1348 (2003)
64.C.H. Wick, P.E. McCubbin, Toxicol. Methods, 9, 245–252 (1999)
65.R. Golmohammadi, K. Valegard, K. Fridborg, L. Liljas, J. Mol. Biol. 234, 620–639 (1993)

10
Structure and Kinetics of Proteins Observed by Small Angle Neutron Scattering
M.W. Roessle, R.P. May
10.1 Introduction
Proteins are the machines of life. These molecules, composed of several hundred to thousands of atoms, are involved in all the processes and reactions inside a living organism. Depending on their functions and tasks, proteins can be classified into two major groups: First the structural proteins, which are responsible for the formation of passive overall structures such as hair fibres or the cytoskeleton of the cell. The second group are the function-related proteins, which facilitate the cell metabolism and regulation. Each protein has at least one three-dimensional structure in which it is stable and active under biological conditions. However, proteins are no rigid bodies, and a stable protein structure can be transformed to other stable states [1, 2]. The transition between the distinct protein conformations is induced thermally or by specific binding of ligands or substrates, and several structural intermediates can be adopted. It is evident that transient intermediates can be more easily identified if one observes a signal that is related to structure, and rate constants can be derived very simply. Simultaneously, direct information about the protein structure during the reaction cycle can be obtained.
10.2 Solution Scattering
Protein structures can be analyzed at high resolution with several methods. X-ray crystallography and nuclear magnetic resonance (NMR) are able to resolve protein structures at the level of atomic sizes (1−3 ˚A), whereas electron microscopy (EM) provides a resolution of about 10−20 ˚A. A wealth of structural and functional information of proteins has been obtained by these methods.
Small angle scattering, a method that allows one to study macromolecular scattering in solution, is an attractive alternative, with in principle no restrictions such as size of the protein or its ability to form single crystals [3,4]. The
188 M.W. Roessle et al.
price to be paid is limited resolution (similar to EM) and the observation of structures that are averaged over all orientations. On the other hand, there is practically no limitation to the composition of the scattering solution and the conditions applied to it, e.g., pressure, temperature, etc.
Since the zero-angle scattering is proportional to the square of the molecular mass of a molecule in solution at a fixed concentration, this parameter allows one to follow the formation of a complex of a macromolecule with another one; the decrease in concentration of the free second molecule (which is proportional to its concentration) diminishes the scattering less than the gain due to square term mentioned before.
The zero-angle scattering cannot be observed directly, due to the direct beam intensity that usually needs to be hidden behind a beamstop, but it can be calculated by extrapolation using the Guinier or Zimm approximations that are valid in a small angular range, in which also the radius of gyration RG of the scattering particles can be obtained. This radius of gyration is equivalent to the radius of inertia in mechanics and is a measure for the elongation of the particles.
Scattering intensities are plotted vs. the momentum transfer Q rather than the scattering angle 2Θ. Q is defined as Q = (4π/λ) sin Θ, where λ is is the neutron wavelength. The Guinier and Zimm approximations are valid in a range of Q < 1/RG.
Beyond this range, the shape of the scattering curves becomes specific for the shape and composition of a particular molecule. For example the particular symmetry of a sphere can be recognized by characteristic minima and maxima of the scattering curve.
In many cases, however, the scattering properties of a molecule are better understood if one transforms the scattering curve I(Q) by a sine Fourier transformation into a pair distance distribution function p(r) according to
|
1 |
0 |
∞ |
|
p(r) = |
|
I(Q) Qr sin Qr dQ. |
(10.1) |
|
2π2 |
Since this transform is infinite with respect to Q, an indirect Fourier transform (IFT) method has been proposed by Glatter [5]. IFT uses a least-squares fit of the amplitudes ci of a set of N Fourier-transformed equidistant B-spline functions φi to the measured scattering curves for obtaining the smoothest p(r) function that is compatible with the scattering data.
Dmax |
|
|
N |
|
I(Q) = 4π 0 |
|
sin Qr |
|
|
p(r) |
Qr |
dQ, where p(r) = i=1 ciφi(r). |
(10.2) |
As can be seen in Eq. 10.2, the integral over r is limited to Dmax, i.e., the system must consist of noninteracting particles with a maximal dimension Dmax at low concentration. Since in general the number of splines used for the fit exceeds the number of free parameters, a regularization procedure is required that will not be discussed here.
10 Structure and Kinetics of Proteins |
189 |
10.2.1 Specific Aspects of Neutron Scattering
Due to the particle and wave duality, neutrons can be used like X-rays as a probe for the internal structure of matter. Contrary to X-rays which are sensitive to the electric field of the atoms, neutrons are deviated by interactions with the nuclei. The strength of this interaction is di erent for every single isotope. In particular, neutrons “see” natural abundance hydrogens (1H or H) completely di erent from deuterons (2H or D; hydrogens that contain an additional neutron in their nucleus). Due to the di erence in mass of hydrogen and deuterium, “heavy water” can be enriched by physical methods. Chemically, hydrogen and deuterium can often be hardly distinguished. This opens the way for the contrast variation method.
In the first instance, this means that the di erent components of biological material, namely proteins, lipids, sugars, and nucleic acids, can be distinguished by neutrons varying the percentage of D2O in a mixture of H2O and D2O. This is due to the fact that the scattering amplitude, also called scattering length, of H is negative and about half as big as that of D or O (oxygen). Lipids, proteins, sugars, and nucleic acids contain di erent and decreasing amounts of hydrogen. If one defines a scattering length density as the sum of all scattering lengths in a volume, divided by that volume, one can observe that the di erent scattering lengths can be matched by specific H2O/D2O mixtures, about 0% D2O for the fatty acid chains, 40% for proteins, about 50% for sugar, and 70% for nucleic acids.
Second, one can grow microorganisms relatively easily, in particular bacteria and yeast, in heavy-water containing media, using either deuterated rich carbon sources or minimal media forcing the microorgnisms to introduce the deuterium by metabolic pathways, and thus produce perdeuterated or partially deuterated proteins, etc. [6, 7]. Perdeuterated proteins even exceed the scattering length of D2O, partially deuterated protein can be tailored such that they are matched by a chosen level of D2O. In Fig. 10.1 the di erent contrast ∆ρ (= ρprotein − ρsolution) of H-proteins and D-proteins is shown.
Finally, the interaction of neutrons with most matter is very weak, allowing one to use bulky sample environment without major e ect on the scattering. The weak interaction also means that biological molecules do not get damaged by irradiation with long-wavelength “cold” neutrons, contrary to what happens with X-rays or electrons.
10.3 Time-Resolved Experiments: Dynamics vs. Steady State
In general, the high resolution techniques mentioned in Sect. 10.2 are able to characterize stable conformations of proteins, for instance the initial conformation before a reaction starts and the final conformation after the reaction
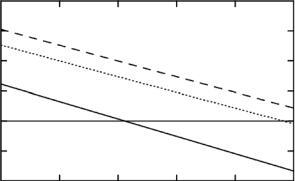
190 M.W. Roessle et al.
) −1 cm
−10 r(10
8
6
4
2
0
−2
−4
0
perdeuterated protein
75% deuterated
natural protein
20 |
40 |
60 |
80 |
100 |
% D2O in H2O/D2O solvent
Fig. 10.1. Contrast ∆ρ of protonated, perdeuterated and 75% deuterated protein as a function of the D2O content in the bu er solution. The scattering length density of protonated (natural) protein is matched by a solvent containing about 40% D2O, that of 75% deuterated protein by nearly pure D2O, while the perdeuterated protein remains always “visible” for neutrons
is finished. But time-resolved methods have to be used in order to trace the reaction between the two conformations. Table 10.1 shows that the conformational changes of proteins can occur on a wide range of time scales and spatial extents. The investigation of these structural changes requires suitable time-resolved methods such as time-resolved crystallography, NMR, or cryo-EM. However, several experimental parameters such as the size of the protein, time scale of the reaction and the triggering of the reaction [8, 9] restrict the use of these techniques. For instance, time-resolved Laue crystallography monitors conformational changes in the nanosecond time range with atomic resolution [10, 11], but is restricted to smaller proteins. Evidently, the crystal lattice itself must be maintained during the observation of the reaction, a condition that is not easily fulfilled, but also the conditions required for obtaining protein crystals may be prohibitive for studying a given reaction. Electron microscopy allows one to investigate larger molecules [12,13], but the solution must be frozen at discrete reaction steps [14]. Finally, NMR proofs time-dependent structural changes in detail, but it is also restricted to small proteins [15].
10.3.1 Protein Motions and Kinetics
The protein can perform work if energy is released by these processes. These protein dynamics occur on di erent time scales with di erent spatial extents. The di erent parameters of protein movements are listed in Table 10.1.

10 Structure and Kinetics of Proteins |
191 |
Table 10.1. The spatial extent and the time scales of movements which occur in proteins (adapted from S. Cusack in [16])
motion |
spatial extent |
characteristic |
|
|
˚ |
time scale (s) |
|
|
(A) |
||
vibrations of bonded atoms |
2–5 |
10−14 |
−10−13 |
elastic vibration of globular regions |
10–20 |
10−12 |
−10−11 |
rotation of side chains at surface |
5–10 |
10−11 |
−10−10 |
relative motions of globular regions |
10–20 |
10−10 |
−10−7 |
allosteric transitions |
5–50 |
10−4−100 |
|
protein folding |
|
10−5−101 |
A special group of function-related proteins are the motor proteins. In contrast to enzymes, which catalyze chemical reactions, working or motor proteins are able to provide mechanical energy. These molecules bind nucleoside triphosphate (NTP) and convert it into nucleoside diphosphate (NDP) by hydrolysis of one phosphate. The released energy is used for processive or continuous motions [17, 18]. During these processive motions mechanical work can be performed. The more general term working protein is used if the protein can provide mechanical work; the motion itself is rather stepwise than continuous [19].
If the transition between the stable protein states requires a cascading process with several structural intermediates, a regulatory process has to be introduced. The simplest regulation is obtained by binding ligands that induce the transition between the inactive and the active conformation to the protein. The ligand acts as an activator for the transition inactive → active conformation, or it can act as an inhibitor for the conversion from the active state to the inactive state. This regulation process is possible for monomeric proteins with one ligand binding site. If the protein is assembled from several identical subunits, a more complicated process takes place. One speaks of an allosteric regulation process, if every subunit of a multimeric protein can bind the ligand, but the whole protein multimer is only functional if all the subunits have bound the ligand. This allosteric regulation is often characterized by special kinetics of the ligand binding [20, 21].
10.3.2 Cooperative Control of Protein Activity
As mentioned above, allosteric regulation is found in multimeric protein assemblies. If these proteins provide more than one ligand binding site, but all binding sites have to bind the ligands before the whole protein is converted into the active state, a regulatory scheme is needed. A so-called allosteric regulation scheme was proposed 1965 by Monod et al. [22]. Its main feature is the enhancement of the a nity for ligand binding after the first ligand is bound. This behavior is called cooperative binding of ligands. The opposite
192 M.W. Roessle et al.
of this behavior, where the binding of a ligand inhibits the binding of other ligands, is called negative or anticooperativity. In large protein assemblies, consisting of several subunits, the allosteric regulation can cause large conformational changes that may even involve the whole protein [23]. In addition, cooperative and anticooperative behavior can occur at the same protein using the same ligand. In these reactions the cooperative binding favors subunits to bind the ligand (which are forced in the tense T state), while it inhibits ligand binding on the other subunits (which stay in the relaxed R state) [23]. The biological advantage of allosteric regulation is that no additional control mechanism for the ligand is needed, because structural changes of the protein upon ligand binding control the reaction. The transition between the T and the R state can occur concerted, sequentially or by a combination of both. Since the analysis of cooperative and allosteric protein activity gives insights into the reaction mechanism and its regulation scheme, the investigation of these processes is of major interest in biophysics and biochemistry.
A typical representative of a cooperatively driven reaction is the chaperonin GroEL from Escherichia coli. Chaperonins assist protein folding and facilitate the repair of misfolded proteins as a part of the shock response of bacteria. For performing this task proteins of the chaperonin family are able to bind denatured proteins as substrates after recognizing hydrophobic patterns on the protein surface [24–26]. GroEL is a multimeric protein that consists of 14 identical subunits with a sevenfold symmetry revealing three di erent subdomains. The entire GroEL is build of two rings of seven subunits, that are stacked together back to back forming the typcial cylindrical shape of class II chaperonins. The regulatory scheme of this large protein assembly (800 kDa) and its 14 ATP binding sides exhibits an remarkable interplay between cooperative and anticooperative nucleotide binding. Only one of the GroEL rings can bind nucleotides, whereas the binding of the same nucleotide type is inhibited at the second ring. This behavior controls the substrate binding as well as the binding of the smaller co-chaperonin GroES to one end of the GroEL cylinder. Figure 10.2 shows the crystal structure and a low resolution model of the GroEL–GroES complex based on SANS data.
Cooperative protein kinetics is often investigated by biochemical steadystate methods. For instance, the steady-state formation rate of molecules in the T state can be measured as a function of ligand concentration using radioactive or fluorescence markers. From this analysis the Hill coe cient, a parameter for cooperative ligand binding can be obtained. Systems far from equilibrium, where the assumption of steady state is no longer valid, need to be studied using transient-state kinetics. For the analysis of a transient reaction mechanism, time-resolved techniques have to be used.
10.4 Protein Kinetic Analysis
by Neutron Scattering Experiments
For kinetic studies of protein–protein interactions the reaction can be triggered by rapidly mixing the reaction partners [27–30], or by rapidly changing