
Neutron Scattering in Biology - Fitter Gutberlet and Katsaras
.pdf6 High-Angle Neutron Fiber Di raction |
101 |
While X-ray fiber di raction is well suited to definitive structural studies of filamentous molecules, isomorphous replacement studies, and to the study of conformational changes in polymer systems, it is usually very di cult to use X-ray data to determine the location of ordered water or hydrogen atoms. Early work [43–45] was successful in using Fourier synthesis methods to investigate features of the B-DNA double helix, but were not very successful in locating water positions around the molecule. Even though DNA samples of this type rarely di ract to better than 3 ˚A resolution, neutron studies have allowed a clear picture of the distribution of ordered water to be obtained, particularly in the A and the D conformations. The recent work that has been carried out on cellulose further emphasizes these points. Despite the availability of X-ray data at atomic resolution, neutron analyses were required to determine the intrachain, interchain, and intersheet hydrogen-bonding interactions responsible for the stability and properties of di erent forms of cellulose.
All of the neutron work described has been carried out on the D19 di ractometer at the ILL, using a thin banana-shaped detector that has an angular aperture of 4◦ × 64◦. Despite being located on a thermal beam at one of the best neutron sources in the world, the di racted neutrons have been sadly under-exploited in the past. The reason for this has been the limited size of the detector. The approach used to collect datasets was, as described earlier, to devise a sequence of detector and sample movements that allowed a contiguous di raction pattern to be constructed in reciprocal space from a large number of individual detector measurements (see Fig. 6.6). At any given instant in time during such an experiment, less than 5% of the di racted neutrons were detected. This has had huge implications for sample size, data collection times and indeed the overall scope of the technique. In particular, such experiments have until now been restricted to the study of polycrystalline fiber samples in which the average di racted intensity per pixel at the detector is considerably higher than it is for samples that give continuous (layer line) di raction.
The D19 di ractometer is about to undergo a major upgrade, as shown in Fig. 6.17. Further details of this project and its significance for chemical crystallography and fiber di raction is given by [46] and [47]. The new instrument will mean that it will be possible to use samples that have hitherto been far too small to study using neutrons. It will also mean that for the first time detailed measurements will be possible of continuous di raction from polymer molecules. This type of di raction predominates in di raction studies of many filamentous viruses such as Pf1 [48], TMV [49], a range of plant viruses [50], as well as drug-DNA and protein-DNA complexes, and it is also a key aspect of changes in ordering that occur during structural transitions [51]. For the work on Pf1 filamentous phage, Mitsch [52] and Langan [53] have demonstrated the feasibility of neutron fiber di raction studies on D19 but were not able to record adequate data as a result of the limited detector size. It should be noted that this is exactly the type of work where the upgraded D19 will have a major impact.
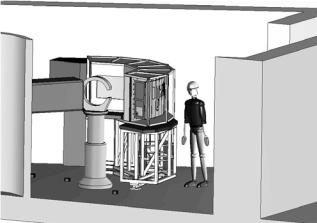
102 V.T. Forsyth et al.
Fig. 6.17. Cartoon showing the new D19 di ractometer at the ILL. The centerpiece of this project is a large area detector that together with upgrades of other aspects of the di ractometer will provide an e ciency gain of 25 on the original instrument
Examples of other work in progress that will benefit from this development are studies of chitin aimed at understanding the involvement of water in the structure, and studies of spider dragline silk that focus on the relationship between the structures of the crystalline and amorphous components of the system [54]. Numerous opportunities also exist for the investigation of key problems in the study of amyloid fibers, where despite considerable progress in the study of amyloid structures in a variety of systems [55–58], many structural questions remain. Other developments in instrumentation suitable for neutron fiber di raction are under way at the Los Alamos LANCSE facility, where the PCS instrument has been built [59, 60], at JAERI which accommodates the BIX monochromatic di ractometers [61], at the FRM-II facility in Munich, at the Rutherford-Appleton ISIS facility in the UK, and at Oak Ridge National Laboratory in Tennessee.
Many of these studies will benefit from and exploit the availability of purpose-designed facilities for the selective and nonselective deuteration of biopolymer systems. Such a facility has been established jointly by the ILL and the EMBL in Grenoble [14] and is now operational with an active and expanding user-driven and in-house research programme. This facility, which will form a key part of the new Partnership for Structural Biology (PSB) between the ILL, the ESRF, the EMBL, and the IBS central facility operators in Grenoble, will have an important impact on the scope of future neutron fiber di raction work on a wide range of important biological polymer systems, either through the provision of samples for which hydrogen incoherent scattering is e ectively eliminated, or samples in which specific parts of the structure have been labelled. A similar laboratory has since been set up at the LANCSE facility at Los Alamos and one is also planned for the Spallation Neutron Source (SNS) in Tennessee. The ability to label biological
6 High-Angle Neutron Fiber Di raction |
103 |
macromolecules in this way has obvious advantages. For crystallographic work and high-angle fiber di raction studies the elimination of hydrogen incoherent scattering has a major impact in terms of the required sample size as well as data collection times and data interpretation. It has been estimated that for a neutron crystallographic study of a typical protein molecule one can expect a gain of a factor of 10 in signal to noise if the protein is perdeuterated. Experiments on perdeuterated myoglobin [62] and on perdeuterated DNA [32] clearly demonstrate these advantages. Selective deuteration is also extremely powerful, as has been demonstrated by Gardner et al. [63] in studies of synthetic polymers. At lower resolution contrast variation can be used in studies where specific parts of multicomponent systems are deuterated while the rest of the structure remains hydrogenated and can be matched out [64].
Acknowledgments
We acknowledge S. Mason and J. Archer at the Institut Laue Langevin, P. Langan at LANSCE, Los Alamos and V. Urban and C. Riekel at the European Synchrotron Radiation Source for valuable discussion. We thank Y. Nishiyama for providing pictures taken from their work on cellulose. We also wish to acknowledge B. Guerard and other members of the ILL Detector Group for all of their e orts in the design and construction of new detector facilities that will soon be installed at the ILL. We acknowledge support from EPSRC under grants GR/R99393/01 and GR/R47950/01 and from the EU under contracts HPRI-2001-50065 and RII3-CT-2003-505925. I.P. acknowledges support from EPSRC and ILL for the provision of a studentship held at Keele University.
References
1.B.K. Vainshtein, Di raction of X-rays by Chain Molecules (Elsevier, Amsterdam 1966)
2.R.D.B. Fraser, T.P. MacRae, in Conformation in Fibrous Proteins (Academic Press, US 1973)
3.R.P. Millane, W. Stroud, Int. J. Biol. Macromol. 13, 202–208 (1991)
4.T. Narayanan, O. Diat, P. Boesecke, Nucl. Instrum. Methods Phys. Res. A 467–468, 1005–1009 (2001)
5.V. Urban, P. Panine, C. Ponchut, P. Boesecke, T. Narayanan, J. Appl. Cryst. 36, 809–811 (2003)
6.C. Riekel, Rep. Prog. Phys. 63, 233–262 (2000)
7.I. Hazemann, M.T. Dauvergne, M.P. Blakeley, F. Meilleur, M. Haertlein,
P.Timmins, A. Van Dorsselaer, A. Mitschler, D.A.A. Myles, A. Podjarny, Acta Cryst. D61, 1413–1417 (2005)
8.W. Fuller, V.T. Forsyth, A. Mahendrasingam, W.J. Pigram, R.J. Greenall,
P.Langan, K. Bellamy, Y. Al-Hayalee, S.A. Mason, Physica B 156/157, 468 (1989)
9.V.T. Forsyth, A. Mahendrasingam, W.J. Pigram, R.J. Greenall, K. Bellamy,
W.Fuller, S.A. Mason, Int. J. Biol. Macromole. 11, 236 (1989)
104V.T. Forsyth et al.
10.P. Langan, V.T. Forsyth, A. Mahendrasingam, W. J. Pigram, S.A. Mason,
W.Fuller, J. Biomol. Struct. Dyn. 10, 489 (1992)
11.A. Rupprecht, Biotechnol. Bioeng. 12, 93 (1970)
12.H. Grimm, H. Stiller, C.F. Majkrzak, A. Rupprecht, U. Dahlborg, (1987), Phys. Rev. Lett. 59, 1780–1783 (1987)
13.H. Grimm, M. Krisch, A. Mermet, V.T. Forsyth, A. Rupprecht, Phys. Rev. E, submitted
14.V.T. Forsyth, D.A.A. Myles, P.A. Timmins, M. Hartlein, Possibilities for the exploitation of biological deuteration in Neutron scattering, in Opportunities for Neutron Scattering in the 3rd Millennium, J. Dianoux (Eds.) (Institut Laue Langevin, Grenoble, 2001), pp 47–54
15.P. Langan, M. Lehmann, C. Wilkinson, G. Jogl, C. Kratky, Acta Cryst. D 55, 51–59 (1999)
16.B. Arhens, M.G. Davidson, V.T. Forsyth, M. Mahon, A.L. Johnson, S.A. Mason, R.D. Price, P.R. Raithby, J. Am. Chem. Soc. 123, 9164–9170 (2001)
17.C.K. Broder, M.G. Davidson, V.T. Forsyth, J.A.K. Howard, S. Lamb, S.A. Mason, Cryst. Growth Des. 2, 163–169 (2002)
18.G. Vives, S.A. Mason, P.D. Prince, P. Junk, J.W. Steed, Cryst. Growth – Des. 3, 699 (2003)
19.S. Detti, V.T. Forsyth, R. Roulet, R. Ros, A. Tassan, K.J. Schenk, Z. Kristallogr. 219, 47–53 (2004)
20.A. Jaworski, W.-T. Hsieh, J.A. Blaho, J.E. Larson, R.D. Wells, Science 238, 773–777 (1987)
21.L.J. Peck, J.C. Wang, Cell 40, 129–137 (1985)
22.S. Wolfl, Biochim. Biophys. Acta 1264, 294–302 (1995)
23.V. Muller, M. Takeya, S. Brendel, B. Wittig, A. Rich, Proc. Natl. Acad. Sci. USA 93, 780–784 (1996)
24.S. Wolfl, C. Martinez, A. Rich, J.A. Majzoub, Proc. Natl. Acad. Sci. USA 93, 3664–3668 (1996)
25.A.G. Herbert, K. Lowenhaupt, J.R. Spitzner, A. Rich, Proc. Natl. Acad. Sci. USA 92, 7550–7554 (1995)
26.T. Schwartz, M.A. Rould, K. Lowenhaupt, A. Herbert, A. Rich, Science 284, 1841–1845 (1999)
27.M.A. Schumacher, B.K. Hurlburt, R.G. Brennan, Nature 409, 215–219 (2001)
28.N. Mizuno, G. Voordouw, K. Miki, A. Sarai, Y. Higuchi, Structure 11, 1133– 1140 (2003)
29.A. Mahendrasingam, V.T. Forsyth, R. Hussain, R.J. Greenall, W.J. Pigram,
W.Fuller, Science 233, 195–197 (1986)
30.V.T. Forsyth, R.J. Greenall, R. Hussain, A. Mahendrasingam, C. Nave, W.J. Pigram, W. Fuller, Biochem. Soc. Trans. 14, 553–557 (1986)
31.I.M. Parrot, V. Urban, K.H. Gardner, V.T. Forsyth, Nucl. Instrum. Methods B, 238, 7–15 (2005)
32.M.W. Shotton, L.H. Pope, V.T. Forsyth, P. Langan, R.C. Denny, U. Giesen, M.-T. Dauvergne, W. Fuller, Biophys. Chem. 69, 85–96 (1997)
33.L.H. Pope, M.W. Shotton, V.T. Forsyth, P. Langan, R.C. Denny, U. Giesen, M.-T. Dauvergne, W. Fuller, Physica B 241–243 1156–1158, (1998)
34.V.T. Forsyth, M.W. Shotton, H. Ye, C. Boote, P. Langan, L.H. Pope, R.C. Denny, Fiber Di r. Rev. 7, (1998) 17–24.
35.M.W. Shotton, L.H. Pope, V.T. Forsyth, R.C. Denny, J. Archer, P. Langan, H. Ye, C. Boote, J. Appl. Cryst. 31, 758–776 (1998)
6 High-Angle Neutron Fiber Di raction |
105 |
36.W. Fuller, V.T. Forsyth, A. Mahendrasingam, Phil. Trans. Roy. Soc. 359, 1237– 1248 (2004)
37.M. Jarvis, Nature 426, 611–612 (2003)
38.P. Langan, Crystallogr. Rev., 11, 125–147 (2005)
39.P. Langan, Y. Nishiyama, H. Chanzy, J. Am. Chem. Soc. 121, 9940–9946 (1999)
40.Y. Nishiyama, H. Chanzy, P. Langan, J. Am. Chem. Soc. 124, 9074–9082 (2002)
41.Y. Nishiyama, J. Sugiyama, H. Chanzy, P. Langan, J. Am. Chem. Soc. 125, 14300–14306 (2003)
42.M. Wada, H. Chanzy, Y. Nishiyama, P. Langan, Macromolecules 37, 8548–8555 (2004)
43.D.A. Marvin, PhD Thesis, University of London (1960)
44.S. Arnott, M.H.F. Wilkins, L.D. Hamilton, R. Langridge, J. Mol. Biol. 11, 391–402 (1965)
45.D.A. Marvin, M. Spencer, M.H.F. Wilkins, L.D. Hamilton, Acta Cryst. 20, 663–669 (1961)
46.V.T. Forsyth, S.A. Mason, J.A.K. Howard, M.G. Davidson, W. Fuller, D.A.A. Myles, Neutron News 12, 10–15 (2001)
47.J.C. Bu et, J.F. Clergeau, R.G. Cooper, J. Darpentigny, A. De Laulany,
C.Fermon, S. Fetal, F. Fraga, B. Gurard , R. Kampmann, A. Kastenmueller,
G.Manzin, F. Meilleur, F. Millier, N. Rhodes, L. Rosta, E. Schooneveld, G.C. Smith, H. Takahashi, P. Van Esch, K. Zeitelhack, Nucl. Instrum. Methods B 554, 392–405 (2005)
48.L.C. Welsh, M.F. Symmons, J.M. Sturtevant, D.A. Marvin, R.N. Perham,
J.Mol. Biol. 283, 155–177 (1998)
49.K. Namba, G. Stubbs, Science 231, 1401–1406 (1986)
50.G. Stubbs, Rep. Prog. Phys. 64, 1389–1425 (2001)
51.M.W. Shotton, L.H. Pope, V.T. Forsyth, P. Langan, H. Grimm, A. Rupprecht, R.C. Denny, W. Fuller, Physica B, 241–243, 1166–1168 (1998)
52.C. Mitsch, PhD Thesis, Cambridge University (1996)
53.P. Langan, Physica B 234–236, 213 (1997)
54.D. Sapede, T. Seydel, V.T. Forsyth, M. Koza, R. Schweins, F. Vollrath,
C.Riekel, Macromolecules 38, 8447–8453 (2005)
55.K. Papanikolopoulou, G. Schoen, V. Forge, V.T. Forsyth, C. Riekel, J.-F. Hernandez, R.W.H. Ruigrok, A. Mitraki, J. Biol. Chem. 280, 2481–2490 (2005)
56.H. Retsos, K. Papanikolopoulou, C. Filipini, C. Riekel, K.H. Gardner, V.T. Forsyth, A. Mitraki, in preparation
57.S.O. Makin, E. Atkins, P. Sikorski, J. Johansson, L.C. Serpell, Proc. Nat. Acad. Sci. 102, 315–320 (2005)
58.U. Baxa, N. Cheng, D.C. Winkler, T.K. Chiu, D.R. Davies, D. Sharma, H. Inouye, D.A. Kirschner, R.B. Wickner, A.C. Steven, J. Struct. Biol. 150, 170–179 (2005)
59.B.P. Schoenborn, P. Langan, J. Synch. Rad. 11, 80 (2004)
60.P. Langan, G. Greene, B.P. Schoenborn, J. Appl. Cryst. 37, 24 (2004)
61.I. Tanaka, K. Kurihara, T. Chatake, N. Niimura, J. Appl. Cryst. 35, 34–40 (2002)
62.F. Shu, V. Ramakrishnan, B.P. Schoenborn, Proc. Natl. Acad. Sci. (USA) 97, 3872–3977 (2000)
63.K.H. Gardner, A. English, V.T. Forsyth, Macromolecules 37, 9654–9656 (2004)
64.W.A. King, D.B. Stone, P.A. Timmins, T. Narayanan, A.A. von Brasch, R.A. Mendelson, P.M. Curmi, J. Mol. Biol. 345, 797–815 (2005)

7
Neutron Scattering from Biomaterials in Complex Sample Environments
J. Katsaras, T.A. Harroun, M.P. Nieh, M. Chakrapani, M.J. Watson, V.A. Raghunathan
7.1 Introduction
The study of materials under di cult environmental conditions (such as high magnetic fields, high pressures, shear, and 100% relative humidity) is by no means straight forward and requires specialized equipment. These conditions may at first seem nonbiological, except for those organisms adapted to extreme environments, but a deeper understanding of biologically relevant materials has been gained from such studies.
In many cases, these experiments are made easier by the fact that neutrons interact weakly, thus nondestructively, with many commonly available materials, like aluminum and its alloys, suitable for the construction of sample cells. Their relatively low cost and useful physical characteristics mean that complex sample environments can readily be accessed with neutrons.
Lipid bilayers in water are perhaps the biologically relevant system most studied under various experimental conditions. The complex phase behavior they exhibit is of general interest to material science, as well as biology. Lipids have been subjected to extremes of temperature and pressure; have undergone detailed hydration studies; and have been aligned under shear and externally applied magnetic fields. The intrinsic properties of neutrons along with the ease of designing and constructing neutron sample environments have enabled us to probe each of these conditions.
In this chapter, we will elucidate, with a variety of recent examples, the power of neutron scattering as a tool to study biologically relevant materials in complex sample environments.
7.2 Alignment in a Magnetic Field
In order to obtain structural details on the atomic scale, the use of a single crystal sample is usually a prerequisite. However, obtaining single crystals of a desired sample is not always possible as many molecules (e.g., deoxyribose nucleic acid (DNA)) do not lend themselves to crystallization. In many such
108 J. Katsaras et al.
cases, however, the use of aligned samples makes it possible to determine certain structural features of the system which can provide su cient information to construct realistic models. Examples of aligned systems providing unique structural information are: DNA [1], plant viruses such as, tobacco mosaic virus (TMV) [2, 3] and papaya mosaic virus (PMV) [4], and various lipid bilayers [5, 6], to name a few.
Over the years, various strategies have been devised to orient samples that have proven either di cult or impossible to crystallize. One such strategy is to align biomolecules in an externally applied magnetic field, B.
The e ect of externally applied magnetic fields on biological systems has been the subject of many studies. In the 1930s, Pauling and Coryell [7] first reported the paramagnetic susceptibility of deoxyhemoglobin and the diamagnetic susceptibility of oxyhemoglobin. More recently, Higashi et al. [8] studied the orientation of erythrocytes in magnetic fields up to 8 T (tesla) and found them to orient with their disk plane parallel to B. Similar behavior was observed with erythrocytes at 4 T [9].
Besides red blood cells, fibrinogen, a plasma protein, is polymerized and aligned in magnetic fields [9]. Maret et al. [10], showed that fragments of high-molecular weight native DNA partially align perpendicular to B and that bases possessing diamagnetic anisotropy are responsible for this alignment. Moreover, Brandes and Kearns [11] demonstrated that liquid crystalline phases of DNA align with the long molecular axes perpendicular to B. Other biological systems that have been aligned in magnetic fields are nematic phases of TMV [12] and membrane complexes such as retinal rods [13] and purple membranes of Halobacterium halobium [14].
With regards to living organisms, frog embryos in a 1 T field [15] exhibited no morphological di erences from unexposed controls, suggesting that magnetic fields have little or no e ect with normal embryonic development. However, a recent study on hemolymph samples from adult bees that had undergone pupal development and emergence in a 7 T field, contained a lower percentage of glucose than controls implying that trehalase enzyme activity is depressed in high magnetic fields [16].
7.2.1 Magnetic Alignment of Lipid Bilayers
It is generally known that lipid membranes orient with their bilayer normals perpendicular to B [17] as shown in (Fig. 7.1a). This is a result of the overall negative diamagnetic anisotropy exhibited by the lipid hydrocarbon chains and their high internal order. Magnetically oriented lipid bilayered micelles, or so-called “bicelles” [18–20], possess great potential as biomimetic substrates in aligning membrane associated peptides and proteins for in-depth structural and dynamic studies. They are composed of a combination of short-chain and long-chain phosphatidylcholines (PCs) such as, dihexanoyl PC (DHPC) and dimyristoyl PC (DMPC), respectively. It is believed that the function of the
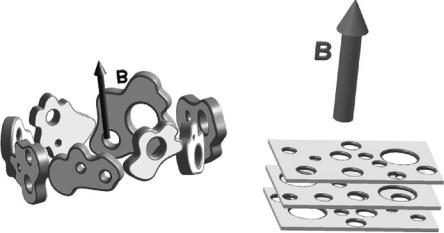
|
7 Neutron Scattering in Complex Sample Environments |
109 |
(a) |
(b) |
|
Fig. 7.1. Cartoon of (a) non-doped DMPC/DHPC mixture (DMPC:DHPC 3.2:1) in the presence of 2.6 T applied magnetic field, B, and a temperature of 315±1 K. Extended bilayered micelles or “finite” lamellar sheets align with their bilayer normals perpendicular to B. (b) The same system as in (a) but doped with Tm3+ ions. In this case, the extended lamellar sheets have their bilayer normals aligned parallel to B. In both the doped and nondoped cases, the bilayers are believed to be perforated [28, 29]. The long-chain DMPC molecules form the bilayer while the short-chain DHPC molecules partition, primarily at the edges of the perforations and the micelles
short-chain lipid is to coat the edges of the relatively small (diameter 10– 100 nm) bilayered micelle, thus protecting the hydrophobic DMPC chains from coming into contact with water. The size of the bicelles is also dependent on the molar ratio of the two lipid species [19, 21].
In a magnetic field, the orientation of DMPC/DHPC mixtures is such that the average bilayer normal, n, is perpendicular to B (Fig. 7.1a). In 1996, Prosser et al. [22] doped mixtures of DMPC/DHPC with paramagnetic ions, such as Tm3+, and found that the orientation of the system altered such that n was now parallel to B (Fig. 7.1b). Compared to nondoped bicelles, the orientation of the lanthanide (e.g., Eu3+, Er3+, Tm3+, and Yb3+) doped bicelles resulted in better resolved NMR spectra. Moreover, the alignment of the nondoped bicelles restricts, due to inhomogeneous broadening of the NMR lines, the size of the membrane associated peptides that can be studied. This limitation is not there in the case of the doped bicelles [23]. Although the DMPC/DHPC bicelle mixture was reconstituted with a number of membrane-associated peptides and proteins [20, 24–26], the morphology of this magnetically alignable substrate was debatable.
In a series of publications, the structures of the lanthanide-doped, DMPGdoped (dimyristoyl phosphatidylglycerol), and nondoped DMPC/DHPC systems (3.2:1, DMPC:DHPC) were reported as a function of temperature
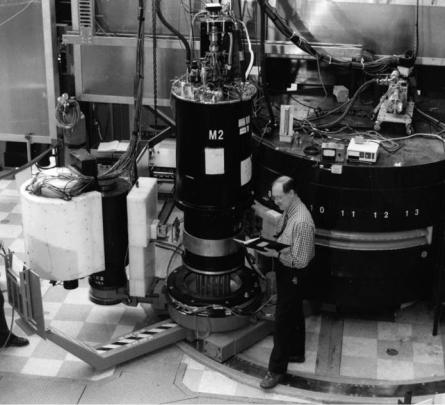
110 J. Katsaras et al.
Fig. 7.2. N5 triple-axis spectrometer with M2 superconducting magnet/cryostat located at the NRU reactor (Chalk River, Canada). 2.37 ˚A wavelength neutrons were selected using the (002) reflection of a pyrolytic graphite monochromator. The M2 magnet/cryostat is a somewhat unique instrument in that it produces a horizontal, rather than a vertical magnetic field
and total lipid concentration [27–29]. Using the C5 and N5 triple-axis spectrometers (Fig. 7.2) located at the National Research Universal (NRU) reactor (Chalk River, Canada) and 2.37 ˚A neutrons, the samples were subjected to a
2.6T horizontal magnetic field (Fig. 7.2), as in the NMR experiment.
At a temperature of 315±1 K, the nondoped DMPC/DHPC mixture sus-
pended in 77 wt% D2O formed a nematic phase, characterized by a single broad peak centered at Q 0.05 ˚A−1 (Q = 2π/d, where d is the lamellar repeat spacing) (Fig. 7.3a), and resulting from bilayered micelles or small bilayer sheets possessing long-range orientational order but lacking positional order [27]. In this phase, the system’s bilayer normals are perpendicular to the magnetic field (Fig. 7.1a) and analogous to a lipid/detergent system studied by X-ray scattering [30]. Upon addition of Tm3+ ions (DMPC:Tm3+, 7.5:1), the system underwent a nematic → smectic transition, as exemplified by the
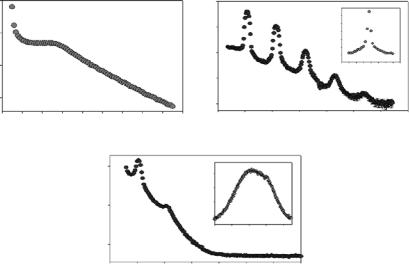
|
|
7 Neutron Scattering in Complex Sample Environments |
111 |
||||||||
106 |
|
|
|
|
105 |
|
|
s |
3 |
|
|
Counts/60s |
|
|
|
|
102 |
|
|
Counts/2 (x10 |
ω (deg.) |
||
|
|
|
|
|
|
|
|
|
) |
2 |
|
|
|
|
|
|
104 |
|
|
3 |
|
||
105 |
|
|
|
|
|
|
|
|
|
1 |
|
|
|
|
|
|
103 |
|
|
|
0 |
|
|
104 |
|
|
|
|
|
|
|
|
|
−8 −4 |
0 4 8 |
103 |
|
|
|
|
101 |
|
|
|
|
|
|
0 |
0.04 |
0.08 |
0.12 |
0.16 |
|
0 |
|
0.10 |
0.20 |
0.30 |
|
(a) |
|
Q [A−1] |
|
|
(b) |
|
|
Q [A−1] |
|
|
|
|
|
105 |
|
s |
|
5 |
|
|
|
|
|
|
|
|
Counts/60 |
|
|
|
|
|
|
||
|
|
Counts/200s |
|
) |
|
|
|
|
|
||
|
|
|
(x10 |
|
|
|
|
|
|
||
|
|
|
|
|
3 |
|
|
|
|
|
|
|
|
104 |
|
|
|
4 |
|
|
|
|
|
|
|
|
|
|
|
|
|
|
|
|
|
|
|
|
|
|
|
0 |
80 |
160 |
|
|
|
|
|
103 |
|
|
|
|
ω (deg.) |
|
|
|
|
|
|
0 |
0.10 |
|
0.20 |
|
0.30 |
|
|
|
(c)Q [A−1]
Fig. 7.3. Scan in Q of (a) DMPC/DHPC system in the absence of Tm3+, at a T
˚−1 |
is indicative of |
|
of 315 K and a 2.6 T field. The broad peak centered at 0.05 A |
3+ |
ions results |
a nematic phase (1D ordering, see Fig. 7.1). (b) The addition of Tm |
|
in a smectic phase (2D order) with well-defined Bragg reflections. The inset to the figure shows that the phase is highly aligned, within a degree, or so, of the applied magnetic field. (c) Removal of the magnetic field results in a less ordered smectic phase, as indicated by the rocking curve (inset), with the lamellar spacing remaining unaltered. For further details the reader is referred to [27]
appearance of well-defined Bragg reflections (Fig. 7.3b), and indicative of a system possessing a well-defined interbilayer spacing, d, of 116 ˚A. Moreover, the system was shown to be highly aligned with the rocking curve having an FWHM of ≤1◦ (inset to Fig. 7.3b). In the absence of an applied magnetic field, the orientation of the system is, for the most part, lost (Fig. 7.3c and inset) while the phase remained unaltered. In summary, the doping of the DMPC/DHPC mixture with Tm3+ ions resulted in the system undergoing a nematic → smectic transition while the magnetic field imparted an alignment to the system [27]. The above-mentioned study was later refined, in the absence of a magnetic field, using small-angle neutron scattering (SANS) [28,29] and whose partial phase diagrams are presented below (Fig. 7.4).
7.2.2 Neutron Scattering in a Magnetic Field: Other Examples
In 1989, Hayter et al. [31], reported on SANS measurements of ferrofluids containing TMV and tobacco rattle virus (TRV). In this case, the nonmagnetic