
Учебники / Hearing - From Sensory Processing to Perception Kollmeier 2007
.pdfContents |
xi |
22 Neural Representation of Frequency Resolution in the Mouse
Auditory Midbrain . . . . . . . . . . . . . . . . . . . . . . . . . . . . . . . . . . . . . . . . . . . . . . . . . .199
MARINA EGOROVA , INNA VARTANYAN, AND GUENTER EHRET
23 Behavioral and Neural Identification of Birdsong under Several
Masking Conditions . . . . . . . . . . . . . . . . . . . . . . . . . . . . . . . . . . . . . . . . . . . . . . . . .207
BARBARA G. SHINN-CUNNINGHAM, VIRGINIA BEST, MICHEAL L. DENT, FREDERICK J. GALLUN, ELIZABETH M. MCCLAINE, RAJIV NARAYAN, EROL OZMERAL, AND KAMAL SEN
Part V Intensity Representation
24 Near-Threshold Auditory Evoked Fields and Potentials are In Line
with the Weber-Fechner Law . . . . . . . . . . . . . . . . . . . . . . . . . . . . . . . . . . . . . . . . . .215
BERND LÜTKENHÖNER, JAN-STEFAN KLEIN, AND ANNEMARIE SEITHER-PREISLER
25 Brain Activation in Relation to Sound Intensity and Loudness . . . . . . . . . . . .227
DAVE LANGERS, WALTER BACKES, AND PIM VAN DIJK
26 Duration Dependency of Spectral Loudness Summation, Measured
with Three Different Experimental Procedures . . . . . . . . . . . . . . . . . . . . . . . . . .237
MAARTEN F.B. VAN BEURDEN AND WOUTER A. DRESCHLER
Part VI Scene Analysis
27 The Correlative Brain: A Stream Segregation Model . . . . . . . . . . . . . . . . . . . . .247
MOUNYA ELHILALI AND SHIHAB SHAMMA
28 Primary Auditory Cortical Responses while Attending
to Different Streams . . . . . . . . . . . . . . . . . . . . . . . . . . . . . . . . . . . . . . . . . . . . . . . . .257
PINGBO YIN, LING MA, MOUNYA ELHILALI, JONATHAN FRITZ, AND SHIHAB SHAMMA
29 Hearing Out Repeating Elements in Randomly Varying Multitone
Sequences: A Case of Streaming? . . . . . . . . . . . . . . . . . . . . . . . . . . . . . . . . . . . . . .267
CHRISTOPHE MICHEYL, SHIHAB A. SHAMMA, AND ANDREW J. OXENHAM
30 The Dynamics of Auditory Streaming: Psychophysics, Neuroimaging,
and Modeling . . . . . . . . . . . . . . . . . . . . . . . . . . . . . . . . . . . . . . . . . . . . . . . . . . . . . . .275
MAKIO KASHINO, MINAE OKADA, SHIN MIZUTANI, PETER DAVIS,
AND HIROHITO M. KONDO
31 Auditory Stream Segregation Based on Speaker Size, and Identification
of Size-Modulated Vowel Sequences . . . . . . . . . . . . . . . . . . . . . . . . . . . . . . . . . . .285
MINORU TSUZAKI, CHIHIRO TAKESHIMA, TOSHIO IRINO, AND ROY D. PATTERSON
32 Auditory Scene Analysis: A Prerequisite for Loudness Perception . . . . . . . . .295
NICOLAS GRIMAULT, STEPHEN MCADAMS, AND JONT B. ALLEN
xii |
Contents |
33 Modulation Detection Interference as Informational Masking . . . . . . . . . . . .303
STANLEY SHEFT AND WILLIAM A. YOST
34 A Paradoxical Aspect of Auditory Change Detection . . . . . . . . . . . . . . . . . . . . .313
LAURENT DEMANY AND CHRISTOPHE RAMOS
35 Human Auditory Cortical Processing of Transitions Between
‘Order’ and ‘Disorder’ . . . . . . . . . . . . . . . . . . . . . . . . . . . . . . . . . . . . . . . . . . . . . . .323
MARIA CHAIT, DAVID POEPPEL, AND JONATHAN Z. SIMON
36 Wideband Inhibition Modulates the Effect of Onset Asynchrony
as a Grouping Cue . . . . . . . . . . . . . . . . . . . . . . . . . . . . . . . . . . . . . . . . . . . . . . . . . . .333
BRIAN ROBERTS, STEPHEN D. HOLMES, STEFAN BLEECK, AND IAN M. WINTER
37 Discriminability of Statistically Independent Gaussian Noise Tokens
and Random Tone-Burst Complexes . . . . . . . . . . . . . . . . . . . . . . . . . . . . . . . . . . .343
TOM GOOSSENS, STEVEN VAN DE PAR, AND ARMIN KOHLRAUSCH
38 The Role of Rehearsal and Lateralization in Pitch Memory . . . . . . . . . . . . . . .353
CHRISTIAN KAERNBACH, KATHRIN SCHLEMMER, CHRISTINA ÖFFL,
AND SANDRA ZACH
Part VII Binaural Hearing
39 |
Interaural Correlation and Loudness . . . . . . . . . . . . . . . . . . . . . . . . . . . . . . . . . |
.359 |
|
JOHN F. CULLING AND BARRIE A. EDMONDS |
|
40 |
Interaural Phase and Level Fluctuations as the Basis of Interaural |
|
|
Incoherence Detection . . . . . . . . . . . . . . . . . . . . . . . . . . . . . . . . . . . . . . . . . . . . . . . |
369 |
|
MATTHEW J. GOUPELL AND WILLIAM M. HARTMANN |
|
41 |
Logarithmic Scaling of Interaural Cross Correlation: A Model Based |
|
|
on Evidence from Psychophysics and EEG . . . . . . . . . . . . . . . . . . . . . . . . . . . . . . |
379 |
|
HELGE LÜDDEMANN, HELMUT RIEDEL, AND BIRGER KOLLMEIER |
|
42 |
A Physiologically-Based Population Rate Code for Interaural Time |
|
|
Differences (ITDs) Predicts Bandwidth-Dependent Lateralization . . . . . . . . . |
389 |
|
KENNETH E. HANCOCK |
|
43 |
A p-Limit for Coding ITDs: Neural Responses and the Binaural Display . . . .399 |
|
|
DAVID MCALPINE, SARAH THOMPSON, KATHARINA VON KRIEGSTEIN, |
|
|
TORSTEN MARQUARDT, TIMOTHY GRIFFITHS, AND ADENIKE DEANE-PRATT |
|
44 |
A p-Limit for Coding ITDs: Implications for Binaural Models . . . . . . . . . . . . |
407 |
|
TORSTEN MARQUARDT AND DAVID MCALPINE |
|
45 |
Strategies for Encoding ITD in the Chicken Nucleus Laminaris . . . . . . . . . . . |
417 |
|
CATHERINE CARR AND CHRISTINE KÖPPL |
|
Contents |
xiii |
46Interaural Level Difference Discrimination Thresholds and Virtual Acoustic Space Minimum Audible Angles for Single Neurons in the
Lateral Superior Olive . . . . . . . . . . . . . . . . . . . . . . . . . . . . . . . . . . . . . . . . . . . . . . .425
DANIEL J. TOLLIN
47 Responses in Inferior Colliculus to Dichotic Harmonic Stimuli:
The Binaural Integration of Pitch Cues . . . . . . . . . . . . . . . . . . . . . . . . . . . . . . . . .435
TREVOR M. SHACKLETON, LIANG-FA LIU, AND ALAN R. PALMER
48Level Dependent Shifts in Auditory Nerve Phase Locking Underlie Changes in Interaural Time Sensitivity with Interaural Level
Differences in the Inferior Colliculus . . . . . . . . . . . . . . . . . . . . . . . . . . . . . . . . . .447
ALAN R. PALMER, LIANG-FA LIU, AND TREVOR M. SHACKLETON
49 Remote Masking and the Binaural Masking-Level Difference . . . . . . . . . . . . .457
G. BRUCE HENNING, IFAT YASIN, AND CAROLINE WITTON
50 Perceptual and Physiological Characteristics of Binaural
Sluggishness . . . . . . . . . . . . . . . . . . . . . . . . . . . . . . . . . . . . . . . . . . . . . . . . . . . . . . . .467
IDA SIVEKE, STEPHAN D. EWERT, AND LUTZ WIEGREBE
51 Precedence-Effect with Cochlear Implant Simulation . . . . . . . . . . . . . . . . . . . .475
BERNHARD U. SEEBER AND ERVIN HAFTER
52 Enhanced Processing of Interaural Temporal Disparities at
High-Frequencies: Beyond Transposed Stimuli . . . . . . . . . . . . . . . . . . . . . . . . .485
LESLIE R. BERNSTEIN AND CONSTANTINE TRAHIOTIS
53 Models of Neural Responses to Bilateral Electrical Stimulation . . . . . . . . . . .495
H. STEVEN COLBURN, YOOJIN CHUNG, YI ZHOU, AND ANDREW BRUGHERA
54 Neural and Behavioral Sensitivities to Azimuth Degrade with Distance
in Reverberant Environments . . . . . . . . . . . . . . . . . . . . . . . . . . . . . . . . . . . . . . . . .505
SASHA DEVORE, ANTJE IHLEFELD, BARBARA G. SHINN-CUNNINGHAM,
AND BERTRAND DELGUTTE
Part VIII Speech and Learning
55 Spectro-temporal Processing of Speech – An Information-Theoretic Framework . . . . . . . . . . . . . . . . . . . . . . . . . . . . . . . . . . . . . . . . . . . . . . . . . . . . . . . . .517
THOMAS U. CHRISTIANSEN, TORSTEN DAU, AND STEVEN GREENBERG
56 Articulation Index and Shannon Mutual Information . . . . . . . . . . . . . . . . . . . .525
ARNE LEIJON
57 Perceptual Compensation for Reverberation: Effects of
‘Noise-Like’ and ‘Tonal’ Contexts . . . . . . . . . . . . . . . . . . . . . . . . . . . . . . . . . . . . . .533
ANTHONY WATKINS AND SIMON MAKIN
xiv |
Contents |
58 Towards Predicting Consonant Confusions of Degraded Speech . . . . . . . . . .541
O. GHITZA, D. MESSING, L. DELHORNE, L. BRAIDA, E. BRUCKERT,
AND M. SONDHI
59 The Influence of Masker Type on the Binaural Intelligibility
Level Difference . . . . . . . . . . . . . . . . . . . . . . . . . . . . . . . . . . . . . . . . . . . . . . . . . . . . .551
S. THEO GOVERTS, MARIEKE DELREUX , JOOST M. FESTEN,
AND TAMMO HOUTGAST
Index . . . . . . . . . . . . . . . . . . . . . . . . . . . . . . . . . . . . . . . . . . . . . . . . . . . . . . . . . . . . . . . . . .559

1 Influence of Neural Synchrony on the Compound Action Potential, Masking, and the Discrimination of Harmonic Complexes in Several Avian
and Mammalian Species
OTTO GLEICH1, MARJORIE LEEK2, AND ROBERT DOOLING3
1Introduction
An important goal of comparative auditory research is to understand the relationship between structure, mechanisms, and function. The ears of mammals and birds are quite different along many dimensions, but the hearing abilities are remarkably similar on a variety of psychoacoustic tasks (Dooling et al. 2000). However, tests involving temporal fine structure now show interesting differences between birds and humans that may permit a more penetrating analysis of the role of structural and mechanical variation among species in the processing of complex sounds.
One major difference between birds and mammals related to substantial differences in cochlear dimensions is the frequency dependent cochlear response delay. In this chapter we analyze how physiological responses and psychoacoustic measures of masking and discrimination may be accounted for by an interaction of species-specific cochlear response delay and the time distribution of harmonic frequencies within these complexes.
2Methods
2.1Stimuli
Stimuli for the studies reviewed here were harmonic complexes with equalamplitude components and component phases selected to produce complexes with monotonically increasing or decreasing frequency across each fundamental period. Complete descriptions of these stimuli may be found in Leek et al. (2005) and in Lauer et al. (2006). These complexes, generally called “Schroeder complexes”, have component frequencies from 0.2 to 5 kHz, and a fundamental frequency of 100 Hz. Variants of the original Schroeder phase
1ENT Department, University of Regensburg, Germany, otto.gleich@klinik.uni-regensburg.de 2National Center for Rehabilitative Auditory Research, Portland VA, Medical Center, USA, Marjorie.Leek@va.gov
3Department of Psychology, University of Maryland, USA, dooling@psyc.umd.edu
Hearing – From Sensory Processing to Perception
B. Kollmeier, G. Klump, V. Hohmann, U. Langemann, M. Mauermann, S. Uppenkamp, and J. Verhey (Eds.) © Springer-Verlag Berlin Heidelberg 2007
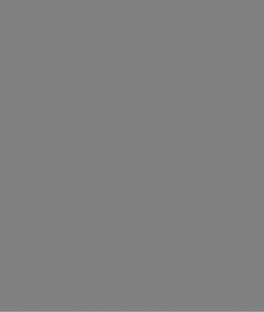
2 |
O. Gleich et al. |
algorithm (Schroeder 1970) include a scalar, C, ranging from ±1.0 in steps of 0.1, that serves to increase or decrease the rate of change of frequency across each fundamental period.
2.2Physiological Measures
The procedures to record evoked cochlear potentials in response to harmonic complexes are described in detail in Dooling et al. (2001). The stimulus waveforms were those shown in Fig. 1 as well as inverted versions to cancel the cochlear microphonic response and isolate the compound action potential (CAP) as a measure of neural synchronization. The stimulus level used for the CAP measurements was set to 70 dBSPL. Physiological data were collected from three budgerigars, two canaries, one zebra finch, four gerbils and two guinea pigs.
2.3Frequency Specific Cochlear Delay
The cochlear delay functions were derived as best fit power functions from scatter plots of published data relating response delay to frequency. These
Fig. 1 Waterfall display of three periods of the waveform for harmonic complexes with a fundamental frequency of 100 Hz created by systematically varying the scalar C in 0.1-steps from −1.0 to +1.0 as indicated by the number at the right side of each waveform. The gray lines in each trace indicate the variation of instantaneous frequency between 0.2 and 5.0 kHz over time. The greater the slope of these lines, the more rapid are the within-period frequency sweeps
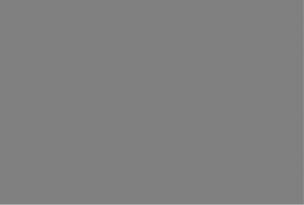
Influence of Neural Synchrony on the Compound Action |
3 |
data come predominantly from auditory nerve fiber recordings in birds (Sachs et al 1974; Gleich and Narins 1988), guinea pig (Palmer and Russell 1986) and gerbil (Schmiedt and Zwislocki 1977) and have been corrected by 1 ms to account for neural delay. Additional bird data came from mechanical measurements of basilar membrane response latency in pigeon (Gummer et al. 1987). Human data are based on the derived ABR data shown in Fig. 3d of Schoonhoven et al. (2001) and frequency specific wave V latency data presented in Table 1 of Donaldson and Ruth (1993), adjusted by 5.3 ms. The resulting best fit power functions relating frequency to cochlear delay for the different species are: human, y = 3.4138x−0.7396; gerbil, y = 0.502x−1.5836; guinea pig, y = 1.6394x−0.7496 and bird, y = 0.6813x−0.6121 with x representing frequency in kHz and y being the delay in ms.
3Results
3.1CAP Amplitude as a Function of Scalar Value
Mean CAP amplitudes are illustrated in Fig. 2 as a function of the scalar value, demonstrating a species specific variation of the CAP amplitude. Negative scalars are on average associated with higher CAP amplitudes than positive scalars, consistent with the notion that upward frequency sweeps tend to “compensate” cochlear delay and cause a higher degree of synchronization compared to downward sweeps (e.g. positive scalars). A prediction for humans, which will be described in the next section, is illustrated as the thick black line in Fig. 2.
Fig. 2 Mean CAP amplitude as a function of scalar value is shown for bird, gerbil and guinea pig, with the number of animals in each group indicated in the legend. The thick black line shows a prediction for CAP amplitude in humans based on the regression line described in the next section
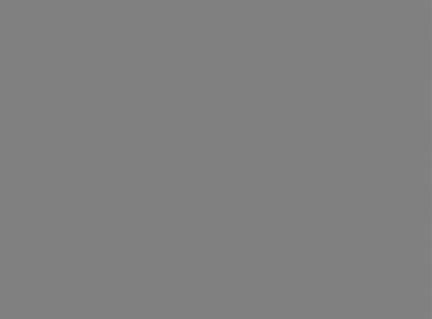
4 |
O. Gleich et al. |
3.2 Cochlear Activation: Interaction of Stimulus Related Frequency Timing and Cochlear Delay
Figure 3 illustrates that cochlear activation over stimulus periods varies considerably between species and scalars. The difference in the duration of cochlear activation by one stimulus period is more pronounced in mammals due to the long response delays at low frequencies, and the difference between positive and negative scalars decreases for scalar values close to 0. A stimulus perfectly compensating cochlear delay would result in synchronized cochlear activation across frequencies and an activation function represented by a vertical line in Fig. 3. A high degree of synchronization should result in a maximized CAP amplitude (Dau et al. 2000). Obviously, none of the harmonic stimuli perfectly compensates cochlear delay in the species studied.
To obtain a quantitative measure of the degree of synchronization we determined the maximum frequency range activated by a single period within a 0.5-ms time window (i.e. around the steepest portion of the cochlear activation functions shown in Fig. 3) as a function of the scalar value for the different species. Since the frequency representation in all species can be regarded as roughly logarithmic, we used the maximally synchronized cochlear region expressed as octaves for this comparison. Figure 4 demonstrates that all species show a maximum synchronization for negative scalars
Fig. 3 Cochlear activation over three stimulus periods for four different scalars in human, gerbil, guinea pig and bird. The dotted line indicates the frequency timing within the stimulus
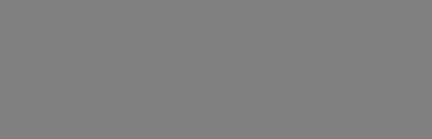
Influence of Neural Synchrony on the Compound Action |
5 |
Fig. 4 The left panel shows the cochlear region responding within a 0.5-ms time window, expressed in octaves, as a function of the scalar value. The right panel plots the CAP amplitude derived from the mean curves in Fig. 2 as a function of the corresponding synchronized octaves of a given scalar shown in the left panel
of −0.1 or −0.2. Overall, birds show a higher degree of synchronization when expressed in octaves. If the frequency scale is converted to physical location on the sensory epithelium the maximally synchronized region is 9 mm for a scalar of −0.2 in humans (almost one third of the organ of Corti) and 2 mm for a scalar of −0.1 in birds (corresponding to 70% of the basilar papilla). The shape of the curves illustrating the synchronized octaves as a function of scalar value (Fig. 4, left panel) is similar to the shape of the mean CAP-amplitude curves (Fig. 2). The right panel in Fig. 4 demonstrates a highly significant correlation between the physiologically determined CAP and the synchronized cochlear region responding within 0.5 ms.
4Discussion
Based on vertebrate cochlear frequency representation, harmonic complexes with within-period frequency sweeps from low to high (negative complexes) can be expected to synchronize neural responses better than those with downward sweeping instantaneous frequencies (positive complexes) because they “compensate” cochlear delays (see also Dau et al. 2000). This is consistent with the general observation that CAP amplitudes for negative scalars tend to be higher than those in response to positive scalars (Fig. 2). Since frequency within a given period of the harmonic complex varies linearly over time (Fig. 1) and the cochlear delay shows a highly non-linear variation with frequency, the interaction results in a complex pattern of cochlear activation over consecutive stimulus periods (Fig. 3). The right diagram in Fig. 4 demonstrates that the analysis of the temporal interaction of an acoustic stimulus and cochlear delay looking at an arbitrarily selected 0.5-ms time window allows reasonable predictions of neural synchronization and CAP amplitude.
The scalar dependent variation of cochlear synchronization (Fig. 4) or CAP amplitude (Fig. 2) differ substantially from the pattern of scalar dependent

6 |
O. Gleich et al. |
variation in the degree of masking reported by Lauer et al. (2006). In an attempt to derive a measure from the cochlear activation analysis (Fig. 2) that might be used to predict the scalar dependent degree of masking, we calculated the time of cochlear activation within one period of the harmonic complex for the frequency range between 2.6 and 3.0 kHz around the signal frequency of 2.8 kHz used by Lauer et al. (2006). The hypothesis is that longer cochlear activation around the signal frequency will cause more masking compared to shorter activation.
Fig. 5 shows that there is a good correlation for positive and negative scalars in birds. Data for negative scalars in humans are similar to data from birds, but masking by positive scaled complexes appears independent of the duration of cochlear activation.
In order to assess whether the differences across species regarding scalar discrimination might be reconciled by taking cochlear activation into account, the data from the Leek et al. (2005) study were replotted as a function of estimates of the difference in total duration of cochlear activation by one period of the standard and the corresponding scaled complex (Fig. 6).
Fig. 5 The diagram shows masked threshold (taken from Lauer et al. 2006) as a function of cochlear activation for the frequency range between 2.6 and 3.0 kHz. Open symbols: negative scalars, filled symbols: positive scalars
Fig. 6 The probability for a correct discrimination as a function of the absolute difference in the duration of cochlear activation between the standard (−1, +1 and 0) and the scaled complexes for humans (left diagram) and birds (right diagram)