
Учебники / Hearing - From Sensory Processing to Perception Kollmeier 2007
.pdf196 |
S.R. Hage et al. |
4.2Other Implications
The Lombard reflex is characterized by an increase in vocal intensity, when the auditory feedback of the vocalizer’s own voice is masked by noise. It can be found in monkeys and humans (Brumm et al. 2004). Furthermore, deafened kittens and monkeys show an increase in vocal intensity, when compared with normal-hearing animals (Romand and Ehret 1984; Talmage-Riggs et al. 1972). This means that the intensity of one’s own voice is automatically up-regulated if it is less than a critical level above the acoustic background. Earlier studies assumed that the Lombard-reflex arc is located in the brainstem, because of its survival in decerebrated cats (Nonaka et al. 1997). With their unique properties of tonically modulating the afferent sensitivity to selfproduced sounds and little or reversed responding to external sounds, the excitatory AVU in the caudal POR are candidates for mediating the Lombard reflex. Their vocalization-related activity could provide the decisive difference in the cochlear responses to self-produced compared to external sounds over a large range of intensities of external sounds.
5Conclusion
The locations and activity patterns of most audio-vocal neurons with suppressed activity to self-produced vocalizations suggest that they are part of the medial olivocochlear system and function to protect the cochlea against overstimulation by own sounds. The location and activity patterns of most audiovocal neurons with increased activity to self-produced vocalization suggest that they are part of the lateral olivocochlear system. Their function is to modulate the activity of cochlear nerve fibers and/or to control the Lombard reflex.
Acknowledgments. The authors thank Ludwig Ehrenreich for technical support and Roland Tammer for medical support. This study was supported by the Deutsche Forschungsgemeinschaft, Ju 181/16-1.
References
Benevento LA, McCleary LB (1992) An immunocytochemical method for marking microelectrode tracks following single-unit recordings in long surviving, awake monkeys. J Neurosci Methods 41:199–204
Brumm H, Voss K, Kollmer I, Todt D (2004) Acoustic communication in noise: regulation of call characteristics in a New World monkey. J Exp Biol 207:443–448
Eliades SJ, Wang X (2003) Sensory-motor interaction in the primate auditory cortex during selfinitiated vocalizations. J Neurophysiol 89:2194–2207
Goldberg RL, Henson OW Jr (1998) Changes in cochlear mechanics during vocalization: evidence for a phasic medial efferent effect. Hear Res 122:71–81
The Olivocochlear System Takes Part in Audio-Vocal Interaction |
197 |
Groff JA, Liberman MC (2003) Modulation of cochlear afferent response by the lateral olivocochlear system: activation via electrical stimulation of the inferior colliculus. J Neurophysiol 90:3178–3200
Grohrock P, Häusler U, Jürgens U (1997) Dual-channel telemetry system for recording vocal- ization-correlated neuronal activity in freely moving squirrel monkeys. J Neurosci Methods 76:7–13
Guinan JJ Jr, Warr WB, Norris BE (1984) Topographic organization of the olivocochlear projections from the lateral and medial zones of the superior olivary complex. J Comp Neurol 226:21–27
Hage SR, Jürgens U, Ehret G (2006) Audio-vocal interaction in the pontine brainstem during self-initiated vocalization in the squirrel monkey. Eur J Neurosci 23:3297–3308
Helfert RH, Aschoff A (1997) Superior olivary complex and nuclei of the lateral lemniscus. In: Ehret G, Romand R (eds) The central auditory system. Oxford University Press, New York, pp 193–258
Huffman RF, Henson OW Jr (1990) The descending auditory pathway and acousticomotor systems: connections with the inferior colliculus. Brain Res Brain Res Rev 15:295–323
Jürgens U (2000) Localization of a pontine vocalization-controlling area. J Acoust Soc Am 108:1393–1396
Jürgens U, Hage SR (2006) Telemetric recording of neuronal activity. Methods 38:195–201 Liberman MC (1988) Response properties of cochlear efferent neurons: monaural vs. binaural
stimulation and the effects of noise. J Neurophysiol 60:1779–1798
Metzner W (1993) An audio-vocal interface in echolocating horseshoe bats. J Neurosci 13:1899–1915
Mulders WH, Robertson D (2001) Origin of the noradrenergic innervation of the superior olivary complex in the rat. J Chem Neuroanat 21:313–322
Mulders WH, Robertson D (2005) Diverse responses of single auditory afferent fibres to electrical stimulation of the inferior colliculus in guinea-pig. Exp Brain Res 160:235–244
Nonaka S, Takahashi R, Enomoto K, Katada A, Unno T (1997) Lombard reflex during PAGinduced vocalization in decerebrate cats. Neurosci Res 29:283–289
Patuzzi RB, Thompson ML (1991) Cochlear efferent neurones and protection against acoustic trauma: protection of outer hair cell receptor current and interanimal variability. Hear Res 54:45–58
Romand R, Ehret G (1984) Development of sound production in normal, isolated, and deafened kittens during the first postnatal months. Dev Psychobiol 17:629–649
Rouiller EM, Capt M, Dolivo M, De Ribaupierre F (1986) Tensor tympani reflex pathways studied with retrograde horseradish peroxidase and transneuronal viral tracing techniques. Neurosci Lett 72:247–252
Suga N, Jen PH (1975) Peripheral control of acoustic signals in the auditory system of echolocating bats. J Exp Biol 62:277–311
Talmage-Riggs G, Winter P, Ploog D, Mayer W (1972) Effect of deafening on the vocal behavior of the squirrel monkey (Saimiri sciureus). Folia Primatol 17:404–420
Tammer R, Ehrenreich L, Jürgens U (2004) Telemetrically recorded neuronal activity in the inferior colliculus and bordering tegmentum during vocal communication in squirrel monkeys (Saimiri sciureus). Behav Brain Res 151:331–336
Thompson GC, Thompson AM (1986) Olivocochlear neurons in the squirrel monkey brainstem. J Comp Neurol 254:246–258
Thompson GC, Igarashi M, Stach BA (1985) Identification of stapedius muscle motoneurons in squirrel monkey and bush baby. J Comp Neurol 231:270–279
Wiederhold ML, Kiang NY (1970) Effects of electric stimulation of the crossed olivocochlear bundle on single auditory-nerve fibers in the cat. J Acoust Soc Am 48:950–965
Wienicke A, Häusler U, Jürgens U (2001) Auditory frequency discrimination in the squirrel monkey. J Comp Physiol A 187:189–195
Xiao Z, Suga N (2002) Modulation of cochlear hair cells by the auditory cortex in the mustached bat. Nat Neurosci 5:57–63
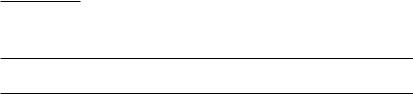
22 Neural Representation of Frequency Resolution in the Mouse Auditory Midbrain
MARINA EGOROVA1, INNA VARTANYAN1, AND GUENTER EHRET2
1Introduction
The theory of frequency analysis of complex sounds in the auditory system is based on the concept of a bank of internal bandpass filters with continuously variable center frequencies. These filters, called critical bands, determine the spectral resolution power of the ear, i.e. the ability to detect and perceive peaks in a sound spectrum separately. Originally, critical bands have been determined in experiments on the perception of tones in noise (Fletcher 1940); therefore they are psychophysical measures. Critical bandwidths (CBs) have been studied in psychophysical tests in humans (e.g. Zwicker and Feldtkeller 1967; Scharf 1970; Moore 1982) and behavioral tests in animals (e.g. Pickles 1975; Ehret 1976; Nienhuys and Clark 1979; Dooling 1980). In these tests, two fundamental properties of critical bands have been established: 1) species-specific dependence of the CBs on their center frequency and 2) intensity independence of the CBs through a wide range of sound intensities.
The starting point of the frequency resolution of the whole auditory system is the frequency-place transformation in the cochlea. For mammals with non-specialized cochleae, CBs cover about equal distances along the basilar membrane (Greenwood 1961, 1990). Hence, the sizes of the CBs of a given species are strongly related to the species cochlear tonotopy. The neural coding of CBs has its first correlate in the frequency tuning of the cochlear nerve fibers without realizing, however, the intensity independence of the CBs (Ehret 1995). How are CBs coded in higher auditory brain centers?
Only for the cat, experimental data on CBs are available for the auditory nerve (Pickles and Comis 1976), the central nucleus of the inferior colliculus (Ehret and Merzenich 1985; 1988) and the primary auditory cortex (Ehret and Schreiner 1997). Compared with behavioral data (Pickles 1975; Nienhuys and Clark 1979) obtained in tests with comparable methods of sound presentation (narrow-band masking), the two main perceptual properties of CBs mentioned above are found in neurons at the midbrain and cortical levels. The midbrain
1Laboratory of Comparative Physiology of Sensory Systems, I.M. Sechenov Institute of Evolutionary Physiology and Biochemistry, RAS, St. Petersburg, Russia, egorova@iephb.ru
2Department of Neurobiology, University of Ulm, Ulm, Germany, guenter.ehret@uni-ulm.de
Hearing – From Sensory Processing to Perception
B. Kollmeier, G. Klump, V. Hohmann, U. Langemann, M. Mauermann, S. Uppenkamp, and J. Verhey (Eds.) © Springer-Verlag Berlin Heidelberg 2007
200 |
M. Egorova et al. |
data lead to the hypothesis that the influence of lateral inhibition flanking the excitatory receptive fields of neurons is important for the determination of the widths of CBs and for their independence of sound intensity (Ehret et al. 1988; Ehret 1995). The major goal of our present studies on the inferior colliculus in the mouse is to test this hypothesis and to determine the relationship between inhibitory receptive fields and neural CBs.
2Methods
Single unit recordings were taken from the central nucleus of inferior colliculus (ICC) of anesthetized (ketamine, xylazine) house mice Mus musculus, hybrids of outbred strain NMRI and feral mice. For every neuron, the conventional single-tone excitatory tuning curve and two-tone response areas (Egorova et al. 2001, 2002) were measured to get the distribution of excitatory and inhibitory response areas in the neuron’s receptive field. Computercontrolled measurements were taken within the whole frequency range of hearing sensitivity of mouse (3–80 kHz) and over a broad range of sound levels: from the units’ tone-response thresholds up to 80 dB above of them, corresponding to a range of −20 to 90 dB SPL. Estimation of the neural CBs was made by simultaneous narrowband white noise masking with constant noise spectral level and variable bandwidth (96 dB/octave slope of the filter). First, a neuron’s response to tone bursts at its excitatory characteristic frequency (CFE) was masked by a continuous broadband noise. Then, the noise bandwidth was narrowed gradually and separately both from the high frequency and the low frequency sides until the tone response appeared again. The noise bandwidth that was just effective in masking the tonal response directly determined the lower and the upper boarders of the critical bands (Vartanian et al. 1999).
3Results and Discussion
3.1Coding of Critical Band Properties
The data presented here are based on recordings from 76 neurons. The main properties of the neuronal CBs in mouse ICC, their dependence on neuron’s CFE and sound intensity, are shown in Fig. 1a,b (Vartanian et al. 1999). Regression analysis (see equation and regression line as solid line in Fig. 1a) showed with high statistical significance (p<0.001) that CBs increased with an increase in the neuron’s CFE. This frequency dependence of neural CBs is very similar to the frequency dependence of psychophysically measured CBs in the mouse (Ehret 1976, line with open circles in
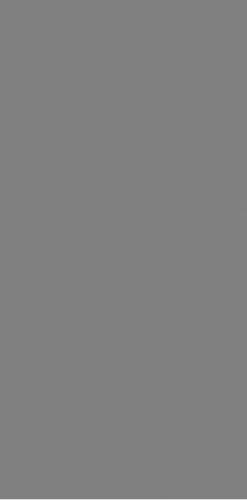
Neural Representation of Frequency Resolution in the Mouse Auditory Midbrain |
201 |
Fig. 1 a Critical bandwidths (left ordinate) measured in single units of the inferior colliculus (closed symbols) and from behavioral tests (line with open symbols; Ehret 1976) of the mouse as a function of the units’ characteristic frequencies or tone frequencies (abscissa). The regression line (solid line) of neural data follows the equation indicated in the figure. The line with closed symbols shows the relationship between cochlea place (in mm from the apex, right ordinate) and the representation of frequencies (abscissa). b Critical bandwidths (ordinate) of single units of the mouse inferior colliculus as a function of the sound pressure level of tones above response threshold (abscissa)
Fig. 1a). Both frequency dependencies of CBs agree with the pattern of frequency representation along the basilar membrane in house mice (Ehret 1975). Hence, the average CFE dependence of CBs reflects the general property of the auditory system which is the dependence of the auditory frequency resolution on the cochlear tonotopy.
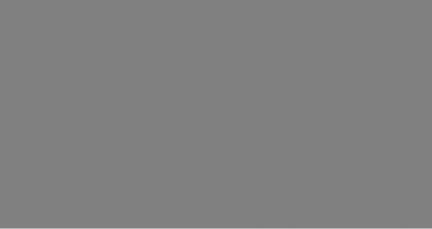
202 |
M. Egorova et al. |
The independence of neural CBs from sound intensity becomes evident in Fig. 1b. On average, neural CBs remain constant over an intensity range of up to at least 70 dB above the neurons’ tone-response thresholds. Thus, the fundamental properties of frequency resolution and critical band formation in hearing are present in the average activity of neurons in the mouse auditory midbrain.
3.2Critical Bands and Neural Excitatory and Inhibitory Receptive Fields
The ICC neurons have been divided into four classes based on the shape parameters of their excitatory frequency response areas, the steepness of slopes on the two sides of the excitatory frequency response areas, and the degree of overlap of excitatory and inhibitory frequency response areas (Vartanian et al. 2000; Egorova et al. 2001). Examples of the receptive fields of neurons of the four classes are shown in Fig. 2. The percentages of neurons in the classes were the following: class I, 35.5%; class II, 31.5%; class III, 29%; class IV, 4%.
Besides one or two excitatory characteristic frequencies (CFE), neurons often display two characteristic frequencies of inhibition, one below (CFLI)
Fig. 2 Examples of receptive field properties of single neurons of the four classes (class I–IV) of the mouse inferior colliculus. Excitatory response areas are indicated by stippling, inhibitory response areas by shades of gray (light gray: partial inhibition, dark gray: total inhibition). Critical bands (solid horizontal lines) are indicated for the different signal levels above the CFE threshold. CFE: neuron’s excitatory characteristic frequency; CFLI: characteristic frequency of inhibition below the CFE; CFHI: characteristic frequency of inhibition above the CFE; CFEL: lowfrequency CFE of class IV neuron; CFEH: high-frequency CFE of class IV neuron; CFCI: characteristic inhibitory frequency of central inhibitory area of class IV neuron. Abscissa: frequency, kHz; ordinate: sound level, dB SPL
Neural Representation of Frequency Resolution in the Mouse Auditory Midbrain |
203 |
and one above (CFHI) the CFE. The position of the CFLI and CFHI relative to the position of the CFE indicate whether or not lateral inhibition starts close to the center of the excitatory response area of a neuron and whether the neuron may be more sensitive to excitatory or inhibitory influences. Usually, class II neurons had the largest inhibitory areas with the strongest inhibition often covering a large part of the excitatory area. Inhibition in class I neurons never extended through the excitatory area. In class III neurons, inhibition was usually weak and inhibitory areas small or only unilateral.
The borders of the critical bands as indicated in Fig. 2 correlated with the shapes of excitatory and inhibitory response areas (Vartanian et al. 2000). CBs always extended at least through part of the excitatory area and often ended in the inhibitory areas. CBs never extended through areas of total inhibition, i.e. through areas in which a tone led to a total inhibition of the neuron’s response to a CFE tone. Thus, areas of total inhibition (dark gray areas in Fig. 2) always marked the limits of the critical band filters. The same relationship between inhibitory response areas and extensions of CBs has been found also in neurons of the cat ICC (Ehret and Merzenich 1988).
3.3Borders of Critical Bands and Characteristic Frequencies of Inhibition
The quantitative evaluation of the borders of CBs in relationship with the characteristic frequencies of inhibition showed highly significant correlations (p < 0.001) in class I–III neurons as indicated by the correlation coefficients
(r) of the linear regression lines in Fig. 3. Data of class IV are not included in this analysis because only few (3) neurons have been recorded. The slopes of the regression lines of the high-frequency borders of the CBs (CBHF) vs the characteristic frequencies of the high-frequency side inhibition (CFHI) are all very close to 1.0 indicating that the high-frequency borders of the neural critical bands are determined by the characteristic frequencies of the highfrequency inhibitory fields. The high-frequency borders of neural CBs (CBHF) can be calculated by subtracting 1.6 kHz (class I), 1.8 kHz (class II) or 3.4 kHz (class III) from the values of the characteristic frequencies of high-frequency inhibitory fields (CFHI). Thus, it is evident that, on average, CBs are strongly restricted in their extension towards high frequencies by the presence of high-frequency lateral inhibition.
The slopes of the regression lines of the low-frequency borders of the CBs (CBLF) vs the characteristic frequencies of the low-frequency side inhibition (CFLI) are close to 1.0 only for class I and class II neurons. For these neurons, the low-frequency borders of CBs (CBLF) can be calculated by adding 3 kHz (class I) or 1.7 kHz to the values of the characteristic frequencies of the lowfrequency inhibitory fields (CFLI). Compared to the high-frequency side, this adding of a frequency range to the CFLI in order to obtain the CBLF indicates that, on average, the inhibition on the low-frequency side is not as strong as on the high-frequency side to stop the extension of the CBs. It is clear,
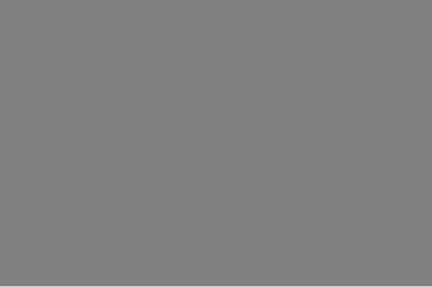
204 |
M. Egorova et al. |
Fig. 3 Correlations between the borders of neural critical bands (CBHF, CBLF) and the characteristic frequencies of inhibition (CFHI, CFLI) of single neurons in the inferior colliculus of the mouse separately for neurons in the three classes (class I–III) and separately for the highfrequency (closed circles) and low-frequency (open circles) CB borders (high-frequency and low-frequency inhibitory areas), respectively. Linear regression lines (solid lines) follow the indicated equations. For further explanations, see text
however, that at least for class I and class II neurons lateral inhibition in the auditory midbrain determines the borders of the CBs. The role of class III neurons with weak inhibitory areas remains to be clarified.
4Conclusions
Fundamental properties of the psychophysical critical band filters, their species-specific frequency dependence and constancy over the wide range of sound intensities are coded in the activity of neurons in the central nucleus of the auditory midbrain. The highly significant correlations between the widths and borders of CBs and characteristics of inhibitory receptive fields of ICC neurons support the hypothesis that CBs are created by the cochlear tonotopy and filtering and are shaped by inhibition at the level of the auditory midbrain to their perceptual properties.
Acknowledgements. Supported by the Volkswagen Foundation (project no. 1/69 589), the DFG (grants to GE), and the Russian Foundation for Basic Research (no. 06-04-48616).
Neural Representation of Frequency Resolution in the Mouse Auditory Midbrain |
205 |
References
Dooling RJ (1980) Behavior and psychophysics of birds. In: Popper AN, Fay RR (eds) Comparative studies of hearing in vertebrates. Springer, Berlin Heidelberg New York, pp 261–288
Egorova M, Ehret G, Vartanian I, Esser KH (2001) Frequency response areas of neurons in the mouse inferior colliculus. I. Thresholdand tuning-characteristics. Exp Brain Res 140:145–161
Egorova MA, Vartanian IA, Ehret G (2002) Neural critical bands and inhibition in the auditory midbrain of house mouse (Mus domesticus). Dokl Biol Sci 382:131–133
Ehret G (1975) Masked auditory thresholds, critical ratios and scales of the basilar membrane of the house mouse (Mus musculus). J Comp Physiol 103:329–341
Ehret G (1976) Critical bands and filter characteristics of the ear of the house mouse (Mus musculus). Biol Cybern 24:35–42
Ehret G (1995) Auditory frequensy resolution in mammals: from neuronal representation to perception. In: Manley GA, Klump GM, Koeppl C, Fastl H, Oekingham H (eds) Advances in hearing research. World Scientific, Singapore, pp 387–397
Ehret G, Merzenich MM (1985) Auditory midbrain responses parallel spectral integration phenomena. Science 227:1245–1247
Ehret G, Merzenich MM (1988) Complex sound analysis (frequency resolution, filtering and spectral integration) by single units of the inferior colliculus of the cat. Brain Res Revs 13:139–163
Ehret G, Schreiner CE (1997) Frequency resolution and spectral integration (critical band analysis) in single units of the cat primary auditory cortex. J Comp Physiol A 181:635–650
Fletcher H (1940) Auditory patterns. Rev Mod Phys 12:47–65
Greenwood DD (1961) Critical bandwidth and the frequency coordinates of the basilar membrane. J Acoust Soc Am 33:1344–1356
Greenwood DD (1990) A cochlear frequency-position function for several species - 29 years later. J Acoust Soc Am 87:2592–2605
Moore BCJ (1982) An introduction to the psychology of hearing. Academic Press, London Nienhuys TGW, Clark GM (1979) Critical bands following the selective destruction of cochlear
inner and outer hair cells. Acta Otolaryngol 88:350–358
Pickles JO (1975) Normal critical bands in the cat. Acta Otolaryngol 80:245–254
Pickles JO, Comis SD (1976) Auditory-nerve-fiber bandwidths and critical bandwidths in the cat. J Acoust Soc Am 60:1151–1156
Scharf B (1970) Critical bands. In: Tobias JV (ed) Foundations of modern auditory theory, vol 1. Academic Press, New York, pp 159–202
Vartanian IA, Egorova MA, Ehret G (1999) Expression of the main properties of critical bands in the neuronal activity of posterior quadrigemini in mice. Dokl Biol Sci 368:437–439
Vartanian IA, Egorova MA, Ehret G (2000) Critical bandwidths of different types of neurons in the mouse auditory midbrain. Dokl Biol Sci 373:364–366
Zwicker E, Feldtkeller R (1967) Das Ohr als Nachrichtenempfänger. Hirzel, Stuttgart
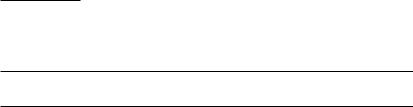
23 Behavioral and Neural Identification of Birdsong under Several Masking Conditions
BARBARA G. SHINN-CUNNINGHAM1, VIRGINIA BEST1, MICHEAL L. DENT2, FREDERICK J. GALLUN1, ELIZABETH M. MCCLAINE2, RAJIV NARAYAN1, EROL OZMERAL1, AND KAMAL SEN1
1Introduction
Many animals are adept at identifying communication calls in the presence of competing sounds, from human listeners communicating in a cocktail party to penguins locating their kin amongst the thousands of conspecifics in their colony.
The kind of perceptual interference in such settings differs from the interference arising when targets and maskers have dissimilar spectrotemporal structure (e.g., a speech target in broadband noise). In the latter case, performance is well modeled by accounting for the target-masker spectrotemporal overlap and any low-level binaural processing benefits that may occur for spatially separated sources (Zurek 1993). However, when the target and maskers are similar (e.g., a target talker in competing speech), a fundamentally different form of perceptual interference arises. In such cases, interference is reduced when target and masker are dissimilar (e.g., in timbre, pitch, perceived location, etc.), presumably by enabling a listener to focus attention on target attributes that differentiate it from the masker (Darwin and Hukin 2000; Freyman et al. 2001).
We investigated the interference caused by different maskers when identifying bird songs. Using identical stimuli, three studies compare (a) human performance, (b) avian performance, and (c) neural coding in the avian auditory forebrain. Results show that the interference caused by maskers with spectrotemporal structure similar to the target differs from that caused by dissimilar maskers.
2Common Stimuli
Targets were songs from five male zebra finches (five tokens from each bird). Three maskers were used that had identical long-term spectral content but different short-term statistics (see Fig. 1): 1) song-shaped noise (steady-state
1Boston University Hearing Research Center, USA, shinn@bu.edu, ginbest@bu.edu, gallun@bu.edu, rn@bu.edu, ozmeral@bu.edu, kamalsen@bu.edu
2Department of Psychology, University at Buffalo, SUNY, USA, mdent@buffalo.edu, mcclain@ buffalo.edu
Hearing – From Sensory Processing to Perception
B. Kollmeier, G. Klump, V. Hohmann, U. Langemann, M. Mauermann, S. Uppenkamp, and J. Verhey (Eds.) © Springer-Verlag Berlin Heidelberg 2007