
Учебники / Hearing - From Sensory Processing to Perception Kollmeier 2007
.pdf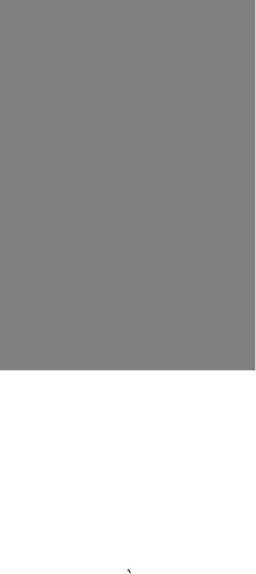
218 |
B. Lütkenhöner et al. |
-
Fig. 2 Intensity dependence of N100m: A amplitude; B latency (Lütkenhoner and Klein, 2007)
The parameter x0 may be interpreted as the N100m threshold: the intensity where the N100m amplitude becomes zero. A least-squares fit based on the intensities below 15 dB (dashed line) resulted in x0= 0.2 dB. Thus, N100m threshold and psychoacoustic threshold agree almost perfectly. For the parameter α we obtained the value 1.19 nAm/dB. A good fit for all intensities is achieved by assuming that the N100m saturates at higher intensities such that its amplitude becomes
s (y) = cy/ c2+ y2 |
(2) |
(gray solid curve in Fig. 2A). For c we derived the value 45.7 nAm. The N100m latency (Fig. 2B) approximately follows the function
L = L3+ Lo :10- q (x - x0 ) /20 |
(3) |
As in Eq. (1), x0 is the threshold extrapolated from the N100m amplitudes. A least-squares fit for the remaining parameters resulted in L∞ = 96.0, L0 =141.9 ms, and q = 0.947 (curve plotted as a gray solid line). The parameter L∞ specifies
Near-Threshold Auditory Evoked Fields and Potentials |
219 |
the N100m latency for very high intensities and may be interpreted as the transmission delay of the system, whereas L0 is the additional delay for a signal of threshold intensity x0.
The following consideration suggests that the additional delay L0 is largely caused by temporal integration. For a system with perfect sound pressure integration, the product of integration time T and sound pressure P is constant: T : P=T0 : P0, where T0 is the integration time for a signal at the threshold P0. With the above terminology, we may write
P / P |
0 |
= 10(x - x0 ) /20 |
(4) |
|
|
|
|
Thus |
|
|
|
T = T0 :10(x - x0 ) /20 |
(5) |
which is basically the second term on the right side of Eq. (3), because the parameter q in that equation is close to 1. At threshold, the integration T0 should correspond to the effective duration of the stimulus. The latter is about 110 ms in our case (counting rise and fall time half). This value is somewhat smaller than the value estimated for L0 in Eq. (3). Nevertheless, in view of the coarseness of the above consideration, the match is surprisingly good.
If we constrain Eq. (3) by letting q =1 and L0=110 ms, a least-squares fit results in the curve plotted as a dashed line in Fig. 2B (the scale on the right refers to the temporal integration effect described by this model). While the fit is excellent at lower intensities, marked deviations are found now at higher intensities. However, the problem could be easily fixed by assuming that the transmission delay is not completely independent of stimulus intensity (slightly shorter delay at higher intensities, which does not appear unreasonable).
3.2Brainstem Auditory Evoked Potential (BAEP)
Figure 3 shows how the time course of the BAEP changes when the intensity of the 4-kHz tone pulse is reduced from 30 to −3 dB SL1. The response is dominated by wave V, which can be recognized at all intensities. Except for the lowest intensities, waves I and III can be recognized as well, but we refrain from considering these waves in more detail.
Figure 4A shows that between 1 and 20 dB SL1 there is a roughly linear relationship between the wave-V amplitude and the intensity in dB, which may again be described by Eq. (1). For x0 we now obtain the value −1.8 dB SL1. Above 20 dB SL1, saturation appears to take effect. Below 1 dB SL1 the response amplitude is larger than predicted by the linear function. This discrepancy is presumably not a measuring error; it appears to indicate that the proposed linear relationship between response amplitude and intensity in dB is not applicable at the limits of audibility.
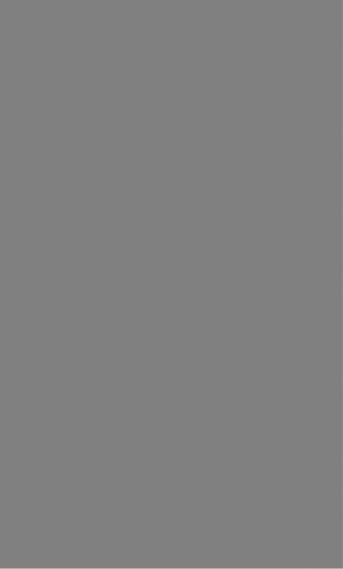
220 |
B. Lütkenhöner et al. |
Fig. 3 Brainstem auditory evoked potential (BAEP) in response to 4-kHz tone pulses (solid lines: ipsilateral recording; dotted lines: contralateral recording). The stimulus intensity was varied between 30 and −3 dB SL1 (sensation level for a single pulse). While BAEP waves I and V can be recognized only at higher intensities, wave V is clearly visible at all intensities. Responses for negative dB values presumably reflect temporal integration (the stimuli were presented not individually, but in a series at intervals of 16 ms)
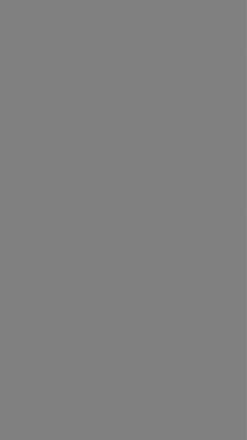
Near-Threshold Auditory Evoked Fields and Potentials |
221 |
Fig. 4 Intensity dependence of: A the amplitude; B the latency of BAEP wave V
Figure 5 offers an alternative view at the data, which appears much more conclusive regarding the lowest intensities. The figure is analogous to Fig. 4A, except that the abscissa represents sound pressure rather than intensity in dB. The figure suggests that between −3 and 9 dB SL1 the response amplitude is a linear function of the sound pressure (dashed line). For comparison, the solid line represents a linear relationship between response amplitude and dB SL1 (corresponding to the solid line in Fig. 4A). The sound pressure was normalized so that the value one corresponds to x0. Threshold extrapolation using the dashed line yields a sound pressure of 0.44. This corresponds to about −8.9 dB SL1(a sound pressure of one corresponds to x0 = −1.8 dB SL1).
Figure 4B shows the intensity dependence of the wave V latency. Between 1 and 10–15 dB SL1, the data could be fitted well by a linear function. At higher intensities the latency changes more slowly than predicted by the

222 |
B. Lütkenhöner et al. |
Fig. 5 Amplitude of wave V as a function of sound pressure. The numbers close to the data points indicate the corresponding intensity in dB SL1
linear function. Irregularities at the lowest intensities are possibly due to the fact that the latency estimation becomes increasingly problematic with decreasing response amplitude.
3.3Psychoacoustic Threshold: Single Pulse vs Sequence of Pulses
BAEP wave V was clearly visible at −3 dB SL1. However this does not mean that we measured a subliminal response. While the dB SL1 scale basically refers to an isolated, single stimulus, the BAEP were measured while presenting a series of stimuli at 16-ms intervals, which gives rise to temporal integration. The sequence was indeed audible at −3 dB SL1, although the sensation was extremely weak. To get an idea of the threshold difference between a single pulse and a series of pulses, supplementary psychoacoustic measurements were done in three subjects. The threshold for two pulses at 16-ms intervals was about −1.6 dB SL1 (roughly consistent with Viemeister and Wakefield 1991), while the threshold for a series of 16 pulses at 16-ms intervals was about −5.5 dB SL1. The latter condition seems to be comparable to the situation during the BAEP measurements.
4Discussion
A logarithmic transformation represents a convenient way to map a continuum spanning many orders of magnitude to a compact scale. This is the reason why the dB scale turned out to be so useful for quantifying physical
Near-Threshold Auditory Evoked Fields and Potentials |
223 |
measures such as sound intensity. Our findings for both wave V of the BAEP and peak N100m of the AEF suggest that, regarding the representation of low sound intensities, the brain also uses a logarithmic transformation.
A roughly linear relationship between the amplitude of the neural response and the logarithm of sound intensity is nothing else than a neurophysiological analogue of the Weber-Fechner law. This does, of course, not mean that our study corroborates the ideas that guided Fechner when deriving the law. Besides that, our data significantly deviate from the law at extremely low intensities (data available only for wave V) and at higher intensities. For wave V, Fig. 5 suggests a more general model. According to that model, the response amplitude is basically a linear function of sound pressure up to intensities of about 9 dB SL1, which is about 18 dB above the threshold that was extrapolated from the response amplitudes. If the intensity increases further, a compressive nonlinearity (similar to Eq. 2) begins to take effect. Taking that model as a basis, a logarithmic scaling analogous to the Weber-Fechner law is merely an approximation which is valid for a relatively limited intensity range.
The threshold extrapolated from the sound-pressure dependence of the response amplitude is almost 6 dB lower than the lowest intensity studied, and more than 3 dB lower than the psychoacoustic threshold estimated for a series of pulses at 16-ms intervals. This implies that the response magnitude at threshold is greater than zero. A corresponding view was proposed for loudness (Buus et al. 1998; Moore and Glasberg 2004; Zwislocki 1965).
References
Buus S, Musch H, Florentine M (1998) On loudness at threshold. J Acoust Soc Am 104:399–410 Fletcher H, Munson WA (1933) Loudness, its definition, measurement and calculation. J Acoust
Soc Am 5:82–108
Klein JS (2006) Auditorisch evozierte Felder im Bereich der Hörschwelle. Dissertation, Medizinische Fakultät der Westfälischen Wilhelms-Universität, Münster
Levitt H (1971) Transformed up-down methods in psychoacoustics. J Acoust Soc Am 49:467–477 Lütkenhöner B, Steinsträter O (1998) High-precision neuromagnetic study of the functional
organization of the human auditory cortex. Audiol Neurootol 3:191–213
Lütkenhöner B, Krumbholz K, Lammertmann C, Seither-Preisler A, Steinsträter O, Patterson RD (2003) Localization of primary auditory cortex in humans by magnetoencephalography. NeuroImage 18:58-66
-
Lütkenhoner B, Klein JS (2007) Auditory evoked field at threshold. Hear Res 228:188–200 Moore BC, Glasberg BR (2004) A revised model of loudness perception applied to cochlear
hearing loss. Hear Res 188:70–88
Relkin EM, Doucet JR (1997) Is loudness simply proportional to the auditory nerve spike count? J Acoust Soc Am 101:2735–2740
Stevens SS (1955) The measurement of loudness. J Acoust Soc Am 27:815–829
Viemeister NF, Wakefield GH (1991) Temporal integration and multiple looks. J Acoust Soc Am 90:858–865
Zwislocki J (1965) Analysis of some auditory characteristics. In: Luce RD, Bush RR, Galanter E (eds) Handbook of mathematical psychology, vol III. Wiley, New York, pp 1–97
224 |
B. Lütkenhöner et al. |
Comment to Lütkenhöner (and Langers) by Chait
In both studies, I wonder how much the responses observed are related to the properties of the acoustic environments in which the listeners operated. In general, does it make sense at all to talk about “perceptual loudness” without considering the specific acoustic context?
In Langers’ fMRI experiment, responses were recorded while listeners were exposed to high intensity machine noise.
In Lütkenhöner’s experiments, stimuli with different intensities were presented in a randomized manner. Since your stimulus set included mostly low intensity stimuli, and since we know that listeners adjust to the properties of their acoustic environments (e.g. Dean et al. 2005) could it be that the particular stimulus set that you used influenced the responses you measure? Specifically, responses to rare high intensity stimuli might be different from those you might have observed if they were less rare. Similarly, might you have observed different responses to low-intensity stimuli if your mean intensity (across stimuli) was still lower?
References
Dean I, Harper NS, McAlpine D (2005) Neural population coding of sound level adapts to stimulus statistics. Nat Neurosci 8:1684–1689
Reply
Your argument does not apply to our BAEP experiment, where the stimulus intensity was typically kept constant for about 15 min. In the MEG experiment, louder stimuli were indeed rare events compared to nearthreshold stimuli, and this fact probably influenced the results to some extent. It is quite conceivable, for example, that a block of stimuli presented at 40 dB SL would have resulted in slightly smaller N100m amplitudes, owing to refractory effects. However, such factors are probably irrelevant near threshold.
Comment by Greenberg
It may be worthwhile to model the variation in magnitude and latency of the brainstem and cortical potentials in terms of excitation patterns rather than stimulus intensity (or other physical parameters). Because there is a systematic shift in latency with frequency as well as with intensity, the two factors may be conflated. This is particularly the case for the cortical M100 MEG response where latency is systematically related to signal frequency
Near-Threshold Auditory Evoked Fields and Potentials |
225 |
(Greenberg et al. 1998). One way to test this hypothesis would be to collect fine-grained parametric data over a variety of intensities and signal frequencies as a means of deriving frequency-intensity latency functions.
References
Greenberg S, Poeppel D, Roberts T (1998) A space-time theory of pitch and timbre based on cortical expansion of the cochlear traveling wave delay. In: Palmer A, Summerfield Q, Rees A, Meddis R (eds) Psychophysical and physiological advances in hearing. Whurr Publishers, London, pp 293–300
Reply
Only the intensity was varied in our experiments. Thus, the data are not suitable for speculations about the effect of stimulus frequency. Previously, we observed a minimum of the N100m latency at tone frequencies between 500 and 1000 Hz (Lütkenhöner et al., 2003), and regarding the lower frequencies this finding is compatible with your idea of a cortical expansion of the cochlear traveling wave delay. But I would like to sound a note of caution. The N100m arises from multiple cortical sources so that the frequency dependence of the N100m latency does not necessarily have a direct counterpart in the activities of the contributing cortical sources. An activity maximum might be observed, for example, at a time when the activity of one source is still rising while the activity of another source is already falling.
References
Lütkenhöner B, Krumbholz K, Seither-Preisler A (2003) Studies of tonotopy based on wave N100 of the auditory evoked field are problematic. Neuroimage 19:935–949
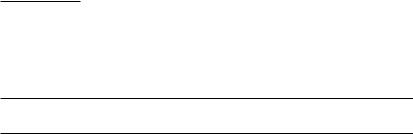
25 Brain Activation in Relation to Sound Intensity and Loudness
DAVE LANGERS1,2, WALTER BACKES3, AND PIM VAN DIJK1,2
1Introduction
In spite of extensive research, still relatively little is known about how sound is processed by the brain and how various sound attributes are neurally represented. Questions remain even regarding very basic attributes like sound level.
In subjects with sensorineural hearing loss, hearing thresholds are elevated over some range of frequencies, due to defects in the inner ear haircells that are required to achieve good sensitivity to soft sounds. At the same time, uncomfortable intensity levels may not have changed notably or may even have decreased. The difference between the hearing threshold and the intensity level of uncomfortable loudness is a measure of the input dynamic range of the ear. A reduction of the input dynamic range is a characteristic of sensorineural hearing loss.
Beside intensity, loudness is the attribute of subjective auditory sensation in terms of which sounds may be ordered on a scale extending from soft to loud. Despite a reduction of intensity range, the loudness range is not reduced in sensorineural hearing loss. A relatively large rise in loudness can then be generated by a relatively small increase in sound intensity. As a result, the relation between loudness and intensity is modified. This phenomenon is known as loudness recruitment (Oxenham and Bacon 2003) and is usually accompanied by other changes related to perception, e.g. a reduced comprehension of speech. As the input to the brain is often permanently changed in people with sensorineural hearing loss, qualitative modifications in the brain activation characteristics as a function of sound intensity and/or loudness may occur. Hence, people with sensorineural hearing loss are of interest in gaining more knowledge on the cortical representation of sound level and potentially for studying plasticity in the auditory system (McDermott et al. 1998).
1Department of Otorhinolaryngology, University Medical Center Groningen, Groningen, The Netherlands, d.r.m.langers@med.umcg.nl
2School of Behavioral and Cognitive Neurosciences, State University Groningen, Groningen, The Netherlands, p.van.dijk@med.rug.nl
3Department of Radiology, Maastricht University Hospital, Maastricht, The Netherlands, wbac@rdia.azm.nl
Hearing – From Sensory Processing to Perception
B. Kollmeier, G. Klump, V. Hohmann, U. Langemann, M. Mauermann, S. Uppenkamp, and J. Verhey (Eds.) © Springer-Verlag Berlin Heidelberg 2007
228 |
D. Langers et al. |
Previous functional magnetic resonance imaging (fMRI) studies have systematically investigated sound level dependent brain activation in normal hearing subjects exclusively. It has been shown that the hemodynamic response signal in the auditory cortex increases with increasing sound level (Jäncke et al. 1998). However, due to the hindrance of acoustic scanner noise as well as the limited signal detection power of fMRI, the determination of sound level dependent activation has been limited to high sound intensity levels over 40 dB sound pressure level (SPL), and often more. Using sparse imaging techniques auditory stimuli can be delivered at low intensities with limited interference from acoustic scanner noise (Hall et al. 1999). Thus, it should be possible to measure brain activation over a much larger intensity range than has yet been done.
For these reasons, an fMRI study was performed to characterize brain responses in a broad intensity level range of 0-70 dB above the hearing threshold for subjects with normal hearing, spanning most of the range that is of daily relevance in life. Also, activation was studied for an equivalent loudness range in subjects with elevated hearing thresholds. In this report, the magnitude of cortical activation will be described as a function of sound intensity level and equivalent loudness level to provide further insights into the relation between brain activation, the strength of the stimulus, and its percept.
2Subject Population and Quality of Hearing
Ten normal hearing subjects (eight male, two female; age 22–61 years, mean 37 years) and ten subjects with high-frequency hearing loss (nine male, one female; age 57–70 years, mean 64 years) were included in this study. Pure tone audiometry was performed to characterize the subject population.
For the normal hearing subjects, hearing thresholds were determined at 7 ± 5 dB hearing level (HL; mean ± standard deviation), averaged over both ears and over frequencies of 0.25–8.0 kHz. In the hearing impaired subjects, average air conduction thresholds for frequencies of 0.25–1.0 kHz equaled 13 ±8 dB HL, and 66 ±11 dB HL for frequencies of 4.0–8.0 kHz. The absolute difference in threshold between the left and right ears averaged over all frequencies was 8±4 dB. Bone conduction thresholds equaled 11 ± 7 dB HL (at 0.25–1.0 kHz) and 59 ± 7 dB HL (at 4.0 kHz; bone conduction thresholds were not determined at 8.0 kHz). Air-bone gaps were smaller than 5 dB on average. Therefore, all impaired subjects suffered from moderately severe symmetrical bilateral sensorineural hearing loss for high frequencies (≥4 kHz), while hearing was normal for low frequencies (≤1 kHz).
To characterize loudness perception at low and high frequencies, highfrequency auditory stimuli were matched in loudness to low-frequency stimuli. In this study, stimuli consisted of frequency modulated (FM) tones with 5-Hz sinusoidal modulations in a spectral range of 0.5–1.0 kHz and 4.0–8.0 kHz, respectively. Because all subjects had normal hearing at low frequencies,