
- •VOLUME 5
- •CONTRIBUTOR LIST
- •PREFACE
- •LIST OF ARTICLES
- •ABBREVIATIONS AND ACRONYMS
- •CONVERSION FACTORS AND UNIT SYMBOLS
- •NANOPARTICLES
- •NEONATAL MONITORING
- •NERVE CONDUCTION STUDIES.
- •NEUROLOGICAL MONITORS
- •NEUROMUSCULAR STIMULATION.
- •NEUTRON ACTIVATION ANALYSIS
- •NEUTRON BEAM THERAPY
- •NEUROSTIMULATION.
- •NONIONIZING RADIATION, BIOLOGICAL EFFECTS OF
- •NUCLEAR MAGNETIC RESONANCE SPECTROSCOPY
- •NUCLEAR MEDICINE INSTRUMENTATION
- •NUCLEAR MEDICINE, COMPUTERS IN
- •NUTRITION, PARENTERAL
- •NYSTAGMOGRAPHY.
- •OCULAR FUNDUS REFLECTOMETRY
- •OCULAR MOTILITY RECORDING AND NYSTAGMUS
- •OCULOGRAPHY.
- •OFFICE AUTOMATION SYSTEMS
- •OPTICAL FIBERS IN MEDICINE.
- •OPTICAL SENSORS
- •OPTICAL TWEEZERS
- •ORAL CONTRACEPTIVES.
- •ORTHOPEDIC DEVICES MATERIALS AND DESIGN OF
- •ORTHOPEDICS PROSTHESIS FIXATION FOR
- •ORTHOTICS.
- •OSTEOPOROSIS.
- •OVULATION, DETECTION OF.
- •OXYGEN ANALYZERS
- •OXYGEN SENSORS
- •OXYGEN TOXICITY.
- •PACEMAKERS
- •PAIN SYNDROMES.
- •PANCREAS, ARTIFICIAL
- •PARENTERAL NUTRITION.
- •PERINATAL MONITORING.
- •PERIPHERAL VASCULAR NONINVASIVE MEASUREMENTS
- •PET SCAN.
- •PHANTOM MATERIALS IN RADIOLOGY
- •PHARMACOKINETICS AND PHARMACODYNAMICS
- •PHONOCARDIOGRAPHY
- •PHOTOTHERAPY.
- •PHOTOGRAPHY, MEDICAL
- •PHYSIOLOGICAL SYSTEMS MODELING
- •PICTURE ARCHIVING AND COMMUNICATION SYSTEMS
- •PIEZOELECTRIC SENSORS
- •PLETHYSMOGRAPHY.
- •PNEUMATIC ANTISHOCK GARMENT.
- •PNEUMOTACHOMETERS
- •POLYMERASE CHAIN REACTION
- •POLYMERIC MATERIALS
- •POLYMERS.
- •PRODUCT LIABILITY.
- •PROSTHESES, VISUAL.
- •PROSTHESIS FIXATION, ORTHOPEDIC.
- •POROUS MATERIALS FOR BIOLOGICAL APPLICATIONS
- •POSITRON EMISSION TOMOGRAPHY
- •PROSTATE SEED IMPLANTS
- •PTCA.
- •PULMONARY MECHANICS.
- •PULMONARY PHYSIOLOGY
- •PUMPS, INFUSION.
- •QUALITY CONTROL, X-RAY.
- •QUALITY-OF-LIFE MEASURES, CLINICAL SIGNIFICANCE OF
- •RADIATION DETECTORS.
- •RADIATION DOSIMETRY FOR ONCOLOGY
- •RADIATION DOSIMETRY, THREE-DIMENSIONAL
- •RADIATION, EFFECTS OF.
- •RADIATION PROTECTION INSTRUMENTATION
- •RADIATION THERAPY, INTENSITY MODULATED
- •RADIATION THERAPY SIMULATOR
- •RADIATION THERAPY TREATMENT PLANNING, MONTE CARLO CALCULATIONS IN
- •RADIATION THERAPY, QUALITY ASSURANCE IN
- •RADIATION, ULTRAVIOLET.
- •RADIOACTIVE DECAY.
- •RADIOACTIVE SEED IMPLANTATION.
- •RADIOIMMUNODETECTION.
- •RADIOISOTOPE IMAGING EQUIPMENT.
- •RADIOLOGY INFORMATION SYSTEMS
- •RADIOLOGY, PHANTOM MATERIALS.
- •RADIOMETRY.
- •RADIONUCLIDE PRODUCTION AND RADIOACTIVE DECAY
- •RADIOPHARMACEUTICAL DOSIMETRY
- •RADIOSURGERY, STEREOTACTIC
- •RADIOTHERAPY ACCESSORIES
RADIOPHARMACEUTICAL DOSIMETRY
HUBERT M.A. THIERENS
University of Ghent
Ghent, Belgium
INTRODUCTION
In nuclear medicine, radiopharmaceuticals are administered to patients for diagnosis or treatment purposes. Each pharmaceutical compound has its specific biodistribution over organs and tissues in the body with related retention times. One method of calculating absorbed dose values delivered internally was developed in the 1960s by the medical internal radiation dose (MIRD) committee of the American Society of Nuclear Medicine (1,2). The original aim was to develop a dosimetry methodology for diagnostic nuclear medicine, but the method can be applied to dosimetry for radionuclide therapy, where the need for an accurate dosimetry is more imperative in view of the high activity levels administered to the patient. The MIRD dosimetry protocol is applied by different international organizations. The International Commission on Radiological Protection (ICRP) has published catalogs of absorbed doses to organs and tissues per unit activity administered, calculated using this dosimetric approach, for most diagnostic radiopharmaceuticals commonly applied (3,4). These tables are established for patients with standard biokinetics of the radiopharmaceutical. For application of the MIRD protocol in the nuclear medicine department when taking into account patient-specific biokinetics a user-friendly computer program called MIRDOSE was developed by Stabin (5). This software has been replaced recently by the authors by an U. S. Food and Drug Administration (FDA) approved program OLINDA (Organ Level Internal Dose Assessment) (6).
By combining well-selected b-emitting radionuclides with disease-specific pharmaceuticals, administration of radiolabeled drugs can provide efficient internal radiotherapy for localized disease as well as for metastatic cancer. As a result, an increasing number of radioactive therapeutic agents are being used in nuclear medicine for the treatment of a large variety of diseases (7–12). For these medical applications of radioactive compounds accurate patientspecific internal dosimetry is a prerequisite. Indeed, the basic goal of the majority of these types of metabolic radiotherapy is to ensure a high absorbed dose to the tumoral tissue without causing adverse effects in healthy tissues. In a curative setting an optimized activity has to be calculated and administered to the patient to ensure the delivery of a predetermined absorbed dose to the tumor resulting in complete tumor control, while minimizing the risk of normal tissue complications. The determination of latter activity necessitates a patient specific dosimetry with respect to drug pharmacokinetics and if possible patient-specific anatomical data.
Nowadays, for most applications patient-specific biokinetics are derived from sequential images after administration of a tracer activity and combined with the MIRD methodology to calculate absorbed doses to target and critical tissues (13). For a more complete dosimetric ana-
RADIOPHARMACEUTICAL DOSIMETRY |
565 |
lysis as in the case of clinical trials, the information from imaging is completed by data obtained from blood sampling and urinalysis. In general, patient anatomy is represented by a standard anthropomorphic phantom (14). However, in a complete patient-specific dosimetry approach the individual patient anatomy is also taken into account and derived from computed tomography (CT) or magnetic resonance imaging (MRI). Three dimensional (3D) absorbed dose estimates are then determined from single-photon emission computed tomography (SPECT) or positron emission tomography (PET) activity data using dose-point kernel convolution methods (15,16), or by direct Monte Carlo calculation (17–20). Dose point kernels describe the pattern of energy deposited by the radiation at various radial distances from a point source. Convolution of the dose point kernel of the considered radionuclide with the activity distribution in the patient results in the absorbed dose distribution in the patient. The general idea of Monte Carlo analysis is to create a model as similar as possible to the real physical system and to create interactions within that system based on known probabilities of occurrence, with random sampling of the probability density functions. For dosimetric applications, differential cross-section data of the interactions of the ionizing particles with matter are used and the path of each particle emitted by the radioactive material is simulated until it is completely stopped. The energy deposited in the medium along the path of the ionizing particles results in the absorbed dose distribution. The advantage of direct calculation by Monte Carlo techniques is that this method allows media of inhomogeneous density to be considered. More information on the application of Monte Carlo techniques in dosimetry can be found in Ref. 21. Several software packages have been devised and validated by different groups for patient specific dose calculations. Typical examples are the 3D-ID code from the Memorial Sloan-Kettering Cancer Center (22), the RMDP-MC code from the Royal Marsden hospital (UK) (23) and the VOXEL-DOSE code from Rouen (France) (24). These programs are based on general Monte Carlo codes also used in other medical applications of ionizing radiation as external beam radiotherapy: EGSnrc (25) and GEANT (26).
Radiolabeled pharmaceuticals are also used in the development of new drugs. Before a drug can be applied to patients in phase I and II clinical trials, different steps have to be taken in the investigation of the toxic effects of the new (radio)pharmaceutical compound. This involves firstly a number of animal studies followed by the administration of the pharmaceutical to a restricted number of volunteers. In general, for these animal and volunteer studies, a radiolabeled formulation of the newly elaborated drug is used with 3H or 14C as radionuclide. Sacrifice of the animals at different time points postadministration and quantitative whole-body autoradiography allow the determination of the biodistribution with metabolite profiling, the retention in the different organs and tissues, and the study of excretion pathways of the pharmaceutical in the animals. In the development of radiopharmaceuticals specifically for nuclear medicine imaging purposes, the new compound can be labeled with gamma-emitting radionuclides and the biokinetics are derived from serial
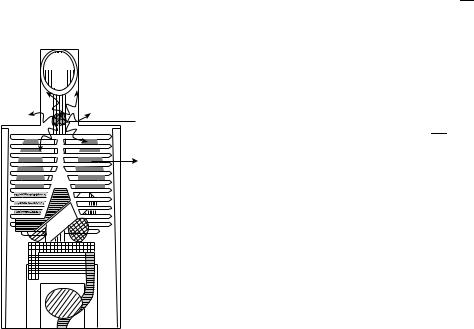
566 RADIOPHARMACEUTICAL DOSIMETRY
imaging of animals. To this end, dedicated (micro)SPECT and (micro)PET systems were constructed (27–29). The animal activity data are extrapolated to humans to determine the maximal activity of the radiolabeled compound allowed to be administered to healthy volunteers. Criterion is here that the effective dose may not exceed the limits for the considered risk category of the volunteers following the ICRP Publication 62 categories (30). In general, the risk category IIa (risk 10 5) with a maximal effective dose of 1 mSv is appropriate for volunteers in testing new drugs. This corresponds to an intermedi- ate-to-moderate level of social benefit for a minor to intermediate risk level for the volunteers. This evaluation procedure necessitates a reliable dosimetry estimate based on the extrapolation of animal activity data to humans. To obtain this dose estimate generally the MIRD formalism is applied.
THE MIRD SCHEMA
Basic Principles and Equations
For patient dosimetry in nuclear medicine diagnostic procedures, the MIRD schema is applied. This formalism is also used systematically for dose calculations in administration of radiolabeled drugs of volunteers in the framework of drugs development.
In the MIRD protocol, organs and tissues in the body with a significant uptake of the radiopharmaceutical are considered as source organs. On the other hand all organs and tissues receiving an absorbed dose are considered as target organs. This is illustrated in Fig. 1 for iodine isotopes as 131I with the thyroid as source organ and the lungs as the target organ on the anthropomorphic phantom developed by Snyder et al. (31). The absorbed dose to a
Source organ
Target organ
Figure 1. Illustration of the MIRD method for the g rays emitted in the decay of iodine isotopes as 131I with the thyroid as source organ and the lungs as target organ in the anthropomorphic phantom developed by Snyder et al. (31).
particular target organ t from a source organ s, Dt s, can be obtained using the following equation (1,2) :
~
Dt s ¼ AsSt s
with ~s is the cumulated activity in the source organ and
A
St s is the mean dose to the target organ per unit cumulated activity in the source organ.
The cumulated activity ~ is the total number of disin-
As
tegrations of the radioactivity present in the source organ integrated over time and expressed in units Bq.s. It depends on the activity administered, the uptake, retention and excretion from the source organ, and the physical decay of the radionuclide. The St s values depend on the decay modes of the considered radionuclide and the sourcetarget geometry. The St s values are tabulated for standard men and children anthropomorphic phantoms (14).
The cumulated activity ~ is the time integral of the
As activity in the source organ AsðtÞ:
Z1
~
As ¼ AsðtÞdt
0
The biological retention in the source organ is generally derived from sequential scintigraphies with a gamma camera. For this, opposing planar views or SPECT are used with a calibrated source in the field of view. Corrections are needed for patient attenuation and scatter of the g radiation. The cumulated activity in the different
source organs ~s allows to calculate the residence time t
A
being the average time the administered activity A0 spends in the considered source organ:
~
t ¼ As
A0
In the MIRDOSE software, the cumulated activity in the different source organs is introduced by the values of the residence time (5).
The mean dose to the target organ per unit cumulated activity in the source organ, St s, is given by the expression:
1 X
St s ¼ mt i Difiðt sÞ
with mt mass of the target organ, Di the mean energy emitted per disintegration for radiation of type and energy i, and wi(t s) the absorbed fraction for radiation of type and energy i. The absorbed fraction wi(t s) is defined as the fraction of the radiation of type i emitted by the source organ s absorbed in the considered target organ t. The Di values are obtained from the decay scheme of the considered radionuclide. The values of the specific absorbed fractions wi(t s)/mt were calculated by Monte Carlo methods (32). The St s values for commonly used isotopes in nuclear medicine calculated in this way for a number of standard anthropomorphic phantoms including children of different ages (14) are tabulated and included in the data base of the MIRDOSE package (5). This procedure assumes a uniform distribution of the activity over the source organs and a standard anatomy of the patient.

|
|
|
RADIOPHARMACEUTICAL DOSIMETRY |
567 |
|
Table 1. Effective Dose Values Per Unit Activity Administered For A Number Of Radiopharmaceuticals Commonly Applied |
|||||
In Nuclear Diagnostics a |
|
|
|
|
|
Radiopharmaceutical |
|
Effective Dose Per Unit Activity Administered (mSv/MBq) |
|
||
|
|
|
|
|
|
|
1 year |
5 years |
10 years |
15 years |
Adult |
|
|
|
|
|
|
18F FDG |
0.095 |
0.050 |
0.036 |
0.025 |
0.019 |
67Ga citrate |
0.64 |
0.33 |
0.20 |
0.13 |
0.10 |
99mTc-DTPA |
0.016 |
0.0090 |
0.0082 |
0.0062 |
0.0049 |
99mTc-HMPAO |
0.049 |
0.027 |
0.017 |
0.011 |
0.0093 |
99mTc-MIBI |
0.053 |
0.028 |
0.018 |
0.012 |
0.0090 |
99mTc-MDP |
0.027 |
0.014 |
0.011 |
0.0070 |
0.0057 |
99mTc-pertechnetate |
0.079 |
0.042 |
0.026 |
0.017 |
0.013 |
99mTc-leucocytes |
0.062 |
0.034 |
0.022 |
0.014 |
0.011 |
111In-octreotide |
0.28 |
0.16 |
0.10 |
0.071 |
0.054 |
123I uptake 35% |
2.05 |
1.08 |
0.51 |
0.34 |
0.22 |
123I-MIBG |
0.068 |
0.037 |
0.026 |
0.017 |
0.013 |
201Tl-chloride |
2.80 |
1.70 |
1.20 |
0.30 |
0.22 |
aSee Ref. 4.
By using the MIRD working procedure, the absorbed doses of the different target organs for frequently used radiopharmaceuticals per unit activity administered were calculated by the ICRP for an adult and children of 1, 5, 10, and 15 years assuming standard biokinetics and were tabulated (3,4). As measure of the radiation burden patient the effective dose E is calculated by summing up the tissue equivalent doses HT using the tissue weighting factors wT as defined in the ICRP 60 publication (33):
X
E ¼ wTHT
T
For the types of radiation emitted by the radionuclides used in nuclear medicine the radiation weighting factor wR is one except for alpha particles, where wR equals 20. In Table 1 the effective dose values per unit activity administered for a number of radiopharmaceuticals commonly applied in nuclear diagnostics under the assumption of standard biokinetics is summarized. Table 1 shows that in diagnostic pediatric nuclear medicine the patient dose is strongly dependent on patient age for the same administered activity. This is mostly due to the change in patient weight. Weight dependent correction factors for the activity to be administered have been calculated to obtain weight independent effective doses (34,35). The concept of effective dose is intended to estimate the risk for late stochastic radiation effects as radioinduced cancer and leukemia in the low dose range, and by this applicable to nuclear medicine investigations for diagnosis. Its value is not representative for the risk for direct deterministic effects as bone marrow depletion in case of therapeutic applications of radiopharmaceuticals.
Microdosimetric Considerations
The S-values commonly applied at the macroscopic level are calculated assuming a uniform distribution of the activity over the source organ and the target being the whole volume of the target tissue. The use of S-values based on these assumptions can lead to erroneous results at the microscopic level in case of self-dose calculation in an organ (target ¼ source) when the isotope distribution is nonuni-
form at the cellular level and particles with range of the order of cellular dimensions are emitted in the decay. This is particularly the case when the radionuclide used is an Auger electron or an alpha emitter. A typical example is the dosimetry of lymphocytes labeled with 99mTc. In Fig. 2, the therapeutic range in soft tissue of the low energy electron groups in the decay of 99mTc is represented and compared to the dimensions of the DNA helix (2 nm) and a lymphocyte (10 mm). Taking into account the range of the large intensity Auger electron groups (2–100 nm) the dose to the DNA in the cell nucleus, which is the biological radiation target in the cell, is strongly dependent if we consider intraor extracellular distribution of a 99mTc radiopharmaceutical. In most radiopharmaceuticals, 99mTc is located extracellularly and the radiation burden from the Auger electrons to the nucleus is very low. In those cases, the cellular dose is due to the 140 keV g-emission and the macroscopic S-values assuming a uniform distribution of the 99mTc activity can be used for the dose calculation. However, in case of intracellular labeling as in the case of labeling of lymphocytes with 99mTc-HMPAO the dose to the lymphocytes due to the Auger electrons is very high, which leads to radiotoxic effects in these cells (36).
For dose assessment in case of intracellular labeling or labeling of the membrane with an Auger electron emitter a microdosimetric approach based on Monte Carlo calculation methods is indicated. In those cases, the MIRD method can be applied at the cellular level for the calculation of the cell nucleus dose from activity uniformly present in the nucleus, the cell cytoplasm or the cell surface using appropriate microscopic St s values tabulated for all radionuclides (37). To cope with nonuniform activity distributions in source organs, determined by PET and SPECT imaging, radionuclide voxel S-values are tabulated for five radionuclides for cubical voxels of 3 and 6 mm (38).
DOSIMETRY FOR RADIONUCLIDE THERAPY
Methodology
In external beam radiotherapy, there is a long tradition in performing treatment planning calculations for each
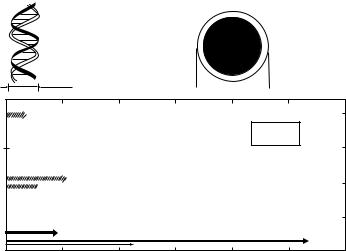
568 RADIOPHARMACEUTICAL DOSIMETRY
Figure 2. The range in soft tissue of the low energy electron groups in the decay of 99mTc, compared to the
dimensions of the DNA helix and a lymphocyte. The latter comparison is relevant for dosimetry in the intracellular labeling of lymphocytes with 99mTc-HMPAO.
Number of electrons per decay
DNA
helix
Lymphocyte
0 002 m
10 m
2.0
99 mTc
1.5
1.0
0.5
0 |
0.01 |
0.1 |
1.0 |
10 |
100 |
1000 |
0.001 |
Range (µm)
individual patient. The dosimetry protocols necessary for metabolic radiotherapy, however, are far more complex than those used in external beam therapy. In fact, the in vivo activity distribution initially is patient-specific and unknown in both space and time. For the determination of the patient specific drug pharmacokinetics, a tracer activity of the radiopharmaceutical is administered to the patient and quantitative imaging at multiple time points is employed to establish patient-specific biokinetics (13). Here, nuclear medicine imaging with proper correction for photon attenuation, scatter, and collimator resolution is needed to obtain the most accurate activity maps possible. The patient-specific biokinetics can then be combined with the MIRD methodology, described above, to calculate absorbed doses to organs and tissues. The MIRDOSE software allows to calculate the self-absorbed dose to a sphere representing the tumor in case of oncological applications. From these dosimetric calculations, the activity of the radiopharmaceutical to be administered to deliver the prescribed absorbed dose level to the considered tissues is then calculated by extrapolation. This approach does not take into account the patient anatomy. Instead, anatomical data of the average male, female, and children of different ages are introduced by anthropomorphic phantoms (14).
More accurate dosimetric calculations require that the individual patient anatomy derived from CT or MRI images is converted into a 3D voxel representation as in external beam radiotherapy. The 3D absorbed dose estimates from the tracer activity administration are then determined from SPECT or PET activity imaging using dose-point kernel convolution methods, or by direct Monte Carlo calculation (15–24). This approach necessitates image fusion between the different imaging modalities lused. The advent of combined SPECT–CT and PET–CT equipment allows a more general application of this complete patient-specific dosimetry (39–43). In this setting, the CT data may be used as an attenuation map, which is an important improvement for accurate quantification (39).
A dosimetry calculation can be useful not only for assessment of the amount of activity to be administered
before radionuclide therapy, but also after the performed radionuclide therapy. First, it is important to verify the predicted absorbed dose distribution. Second, the dosimetry results of a patient population can be combined with the outcome of the therapy to analyse the dose-response of the radionuclide therapy and to make changes in the therapy protocol when necessary (e.g., the predetermined target dose level). As was the case for the pretherapy calculation of the administered activity, posttherapy dosimetry can be performed at different levels of sophistication.
Dosimetry is not only important in the framework of therapy prediction, but also in the dose assessment of organs at risk. In radiopeptide therapy, the kidneys are the dose-limiting organ (44,45). Radiopeptides are cleared physiologically via the kidneys. Most peptides are cleaved to amino acids as metabolites in the kidneys with a high and residualizing uptake in the tubular cells. Damage to the kidneys induced by the radiolabeled metabolites can cause nephropathy after therapeutic application of radiopeptides (46). Application of basic amino acids can reduce the renal accretion of radiolabeled metabolites and the kidney dose (47).
Dosimetry of Radioiodine Therapy for Thyrotoxicosis
The most common application of radionuclide therapy is treatment of hyperthyroidism as observed in Graves’ disease or Plummer’s disease (toxic nodular goiter) by oral administration of 131I. The rationale behind dosimetry for this kind of treatment is that at long-term hypothyroidism may be the outcome for patients treated with radioiodine and that the incidence of this inverse effect is higher with an earlier onset for patients treated with higher activities (48). A large variation exists in the literature on the value of target dose to be delivered to the hyperthyroid tissue to become euthyroid. Howarth et al. (49) reported that doses of 60 and 90 Gy cured 41 and 59 % of patients after 6 months. Guhlmann et al. (50) cured hyperthyroidism in 83 % of patients at 1 year post-treatment with a dose of 150 Gy.AccordingtoWillemsenetal. (51)hyperthyroidismis

eliminated in all patients 1 year post-treatment with a doseof 300 Gy, but at this high dose level 93% of patients became hypothyroid.
For dose calculation in general an adapted version of the Quimby-Marinelli formula (52) has to be used
A |
mCi |
Þ ¼ |
6:67 Dose ðcGyÞ mass ðgÞ |
ð |
|
T1=2eff ðdaysÞ % uptakeð24 hÞ |
Application of this protocol for individual patient dosimetry necessitates the determination of the following important variables: percentage uptake 24 h after administration, effective half-life of the radioiodine, and mass of the thyroid gland. For uptake and kinetics assessment, serial scintigraphies or probe measurements of the patient’s thyroid after administration of a tracer dose have to be performed. This approach assumes that the kinetics of a tracer and a therapeutical amount of administered activity are the same. According to some authors, a pretherapeutic tracer dose may induce a stunning effect limiting the uptake of the therapeutic activity in the thyroid afterward (53). The thyroid mass is generally determined by the pretherapeutic scintigraphy, by ultrasonography or by MRI (54). A 124I PET image also allows measurement of the functioning mass of the thyroid (55). Dosimetry protocols exist based on only a late uptake measurement at 96 or 192 h after tracer activity administration (56). A thorough discussion of the activity to be administered and the dosimetry protocol to follow can be found in Refs. 57,58.
Dosimetry of Radioiodine Therapy for Differentiated Thyroid Cancer
Radioiodine is also administered frequently to patients for differentiated thyroid cancer to ablate remnant thyroid tissue in the early postoperative period, for locoregional recurrences, and for distant metastases. Although most centers administer standard activities, typically 2.8– 7.4 GBq (75–200 mCi), because of the practical difficulties to determine the target absorbed dose, absorbed dose-based protocols are also applied (59). For the calculation of the activity to be administered to give a predetermined tumor absorbed dose protocols as for thyrotoxicosis treatment described earlier are used. As predetermined absorbed dose-to-remnant thyroid tissue a value of 300 Gy is considered to be sufficient (60). For treatment of metastases lower doses giving a complete response have been reported: 85 Gy (61) and 100-150 Gy (62). This approach necessitates determination of the remnant mass of thyroid tissue or metastases by the methods described earlier, which is now more difficult in practice. This introduces in general a large uncertainty on the activity to be administered to ensure the desired dose to the target tissue. Also, the radioiodine kinetics with the 24 h uptake and the effective half-life has to be determined for the patient by administration of a tracer dose. Because of the relatively high activities necessary for quantitative imaging of the target thyroid tissue for this application (at least 37 MBq-1 mCi 131I) complication of the therapy by stunning introduced by the tracer activity mentioned earlier, is more critical here. Because of this and the inaccuracy in the target mass
RADIOPHARMACEUTICAL DOSIMETRY |
569 |
determination, dosimetry protocols based on target dose levels remain difficult for treatment of differentiated thyroid cancer. The 124I PET imaging allows a more exact in vivo determination of iodine concentration and volume determination. By using this method, radiation doses to metastases ranging between 70 and 170 Gy were delivered to the lesions (63).
Instead of target absorbed dose-based protocols, dosimetry protocols based on the largest safe approach are also applied. This approach based on the dose to the critical tissues allows the administration of the maximum possible activity to achieve the maximum therapeutic efficacy. Application of this method necessitates serial total body scintigraphy after the administration of a tracer dose. The dose to the bone marrow, the lungs, and the thyroid tissue or metastases is then calculated by the MIRD formalism. From the absorbed doses obtained by the tracer activity imaging the amount of activity giving the maximal tolerable absorbed dose to the critical tissues is calculated. It has been generally accepted that the activity that delivers 2 Gy whole body dose as a surrogate for the bone marrow dose with a whole body retention < 4.44 GBq (120 mCi) at 48 h postadministration is safe with respect to bone marrow suppression (64). In some departments, the tolerable dose level of the bone marrow in radioiodine treatment of patients with metastatic differentiated thyroid cancer is even increased to 3 Gy based on the LD5/5 data of external beam radiotherapy with LD5/5 being the dose for the red marrow giving a 5% risk of severe damage to the bloodforming system within 5 years after administration (62). Very high activities of 131I in the range 7.4–37.9 GBq (200– 1040 mCi) are then administered for treatment of metastases. In a retrospective study of patients treated with this protocol over a period of 15 years, transient bone marrow depression with thrombopenia and leukopenia was observed recovering after a few weeks (62). No permanent damage was observed. In 10% of the patients the doselimiting organ were the lungs for which a limit of 30 Gy
was adopted from LD5/5 data.
A recent review of the evolving role of 131I for the treatment of differentiated thyroid carcinoma can be found in Ref. 9. Preparation of patients by administration of recombinant human thyroid-stimulating hormone (rhTSH) may allow an increase in the therapeutic radioiodine activity while preserving safety and tolerability (65). As side effects of the 131I therapy, impairment of the spermatogenesis in males (66) and earlier onset of menopause in older premenopausal women (67) are reported. With respect to pregnancy, it is recommended that conception be delayed for 1 year after therapeutic administrations of 131I and until control of thyroid hormonal status has been achieved. After this period there is no reason for patients exposed to radioiodine to avoid pregnancy (68).
Dosimetry of 131I-MIBG Therapy
Another radionuclide therapy application for which the importance of patient-specific dosimetry is generally accepted is treatment of pediatric neuroblastoma patients with 131I-MIBG. Neuroblastoma is the most common
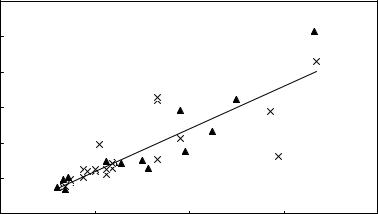
570 RADIOPHARMACEUTICAL DOSIMETRY
extracranial solid tumor of childhood with an incidence of 1/ 70000 children under the age of 15 (69). Neuroblastoma cells actively take up nor-adrenalin via an uptake-1 system. The molecule meta-iodo benzyl guanidine (MIBG), radiolabeled with 131I, has a similar molecular structure, uptake, and storage in the cell as nor-adrenalin. Since 1984, 131I-MIBG has been used therapeutically in neuroblastoma patients (70,71). Aside from the tumor, 131I-MIBG is also taken up in the liver, heart, lungs, and adrenal glands. The bladder is irradiated by the metabolites of 131I-MIBG. For patient dosimetry the largest safe dose approach is applied in 131I-MIBG therapy with bone marrow as dose limiting organ. In practice, the whole body absorbed dose is also used in this setting as an adequate representation or index of bone marrow toxicity. Most treatment regimens consider the maximal activity to be administered limited by rendering a bone marrow dose of 2 Gy. Prediction of whole body doses is based on a pretherapeutic administration of 123I- MIBG. In Fig. 3, predicted whole body doses based on pretherapeutic 123I-MIBG scintigraphies are compared to doses received by patients after 131I-MIBG therapy (72). The received dose values were derived from post-therapy scans. This figure shows also that in the case of repeated therapies pre-therapy scans do not need to be repeated before each therapy except when the biodistribution of 131I-MIBG is expected to change rapidly (e.g., for patients where bone marrow invasion is present). It has also been shown that the accuracy of whole body dosimetry improves when half-life values of tracer and therapy radionuclides are matched (73).
In 131I-MIBG therapy, protocols with administration based on fixed activity per unit mass protocols are also applied. Matthay et al. (74) reported on dosimetry performed in a dose escalation study of patients treated with 131I-MIBG for refractory neuroblastoma with a fixed activity per unit mass ranging from 111 to 666 MBq kg 1 (3–18 mCi kg 1). Patients treated with a specific activity < 555 MBq kg 1 did not require hematopoietic stem cell support, while this was necessary for one-half of the patients treated with a higher specific activity. The median
whole body dose of the group of patients requiring hematopoietic stem cell support was 3.23 Gy (range 1.81– 6.50 Gy) while for the other patients the median dose was 2.17 Gy (range 0.57–5.40 Gy).
In order to improve the results of 131I-MIBG therapy for patients refractive of extensive chemotherapy treatment, a high activity 131I-MIBG schedule is now being used in combination with topotecan as radiosensitizer for the therapy of neuroblastoma in a controlled ESIOP (European International Society of Pediatric Oncology for Neuroblastoma) study protocol (75). The aim here is to administer in two fractions the amount of activity needed to reach a combined total body dose of 4 Gy. These kinds of high doses will inevitably invoke severe side effects, thus frequently necessitating hematopoietic stem cell support and even bone marrow transplantation. However, a contemporary oncological department is well equipped to deal with this kind of treatments. The first amount of 131I-MIBG activity is administered based on a fixed activity per unit body mass (444 MBq kg 1–12 mCi kg 1) protocol. Total body dosimetry is carried out using serial whole body scintigraphies after the first administration. Radionuclide kinetics are followed by a whole body counter system mounted on the ceiling of the patient’s isolation room. These dosimetry results are then used to calculate the activity of the second administration of 131I-MIBG giving a total body dose of 4 Gy over the two administrations. The first results of this study indicate that in vivo dosimetry allows for an accurate delivery of the specified total whole body dose and that the treatment schedule is safe and practicable (75). The approach has now to be tested for efficacy in a phase II clinical trial.
Dosimetry in Radioimmunotherapy
In general, red marrow is the dose-limiting tissue in nonmyeloablative and lung for myeloablative radioimmunotherapy (RIT). Administration protocols are applied based on absorbed-dose values of the dose-limiting tissue and on an activity per body weight basis. Typical examples
Figure 3. Correlation between the whole body dose estimate based on 123I-MIBG pretherapy scans and the dose derived from 131I-MIBG posttherapy scans in patients treated for neuroendocrine tumors. The triangles represent the data of the first therapies, the crosses the data of retreatments. The straight line is the result of a linear regression to all data (R2 ¼ 0.73).
Dose (Gy) based on 131I-MIBG post therapy scans
3
2.5
2
1.5
1
0.5
0
0 |
0.5 |
1 |
1.5 |
2 |
Dose (Gy) based on 123I-MIBG pre therapy scans
of these protocols are the 131I-labeled anti-CD20 antibody, tositumomab (Bexxar; Glaxo-SmithKline) (76) and the 90Y-labeled anti-CD20 ibritumomab tiuxetan (Zevalin; Biogen Idec) (77), respectively. These radiolabeled antibodies are used for treatment of non-Hodgkin’s lymphoma. The choice for an activity-based protocol for the 90Y-labeled antibody is based on the lack of correlation between absorbed dose and toxicity in the early studies. The explanation for the absence of a dose-response rela-
tionship can be found in different sources. In contrast to 131I, 90Y is a pure b-emitter, and 90Y kinetics have to be
derived from surrogate 111In imaging. Another point is that prior treatment of these patients and the bone marrow reserve have a strong effect on the bone marrow toxicity in this case. As patients undergoing RIT have been treated previously by chemotherapy, the impact of such prior therapy on the hematopoietic response to the RIT is important.
Although the necessity of patient-specific dosimetry is questionable in some applications of RIT where the doseresponse observations for toxicity are poor, there is a general agreement that complete radiation dosimetry is necessary for each new application of a radiolabeled antibody in phase I and most probably also in phase II studies especially for safety reasons (78). An important argument for absorbed dose driven protocols in clinical phase I trials is that many patients are treated below the biologically active level due to the interpatient variability in activity based administration protocols. This implies data difficult to interpret in antitumor response and toxicity.
In view of the central role of red marrow toxicity in RIT methodologies, bone marrow dosimetry got already a lot of attention in the literature (79–85). In general, methods based on imaging as described in the section on 131I-MIBG therapy are used. Also, approaches to calculate the bone marrow dose based on blood activity measurements have been described, but these methods yield only reliable results when the activity does not bind specifically to blood or marrow components including tumor metastases in the marrow (79). By assuming rapid equilibrium of radiolabeled antibodies in the plasma and the extracellular fluid of the red marrow, a red marrow/blood concentration ratio of 0.3–0.4 can be derived. All red marrow dosimetry performed up to now uses a highly stylized representation of the red marrow over the body. More detailed representations are being generated especially for Monte Carlo calculations enhancing accuracy and reliability of the bone marrow doses (86).
Several studies have investigated the relation between the tumor dose and response especially in RIT of nonHodgkin’s lymphoma (87–89) but the results are negative. Possible explanations are the therapeutic effect of the antibody, different confounding biological factors and the accuracy of tumor dosimetry. Here, standardization of data acquisition as presented in MIRD pamphlet No. 16 (13) may help in dose-response investigations. As discussed earlier in the section on 131I-MIBG therapy, a full patient-specific 3D dosimetric approach with imaging data from the combined SPECT–CT systems will improve substantially the accuracy of the tumor dosimetry results.
RADIOPHARMACEUTICAL DOSIMETRY |
571 |
DOSIMETRY IN THE DEVELOPMENT OF NEW DRUGS
For the study of the absorption, metabolism, and excretion pathways of new drugs a 3H- or 14C-radiolabeled formulation of the drug is administered to healthy volunteers. A dosimetric evaluation of the radiation burden of the volunteers based on animal biodistribution, retention, and excretion data is necessary and presented to an ethical committee before the radiolabeled drug can be administered. This procedure has to ensure that the effective dose will not exceed the limits for the considered risk category of the volunteers according to the ICRP publication 62 categories (30). For testing new drugs mostly a risk category IIa (risk 10 5) is adopted corresponding to a maximal effective dose of 1 mSv. Based on this criterion, the activity to be administered is calculated from the dosimetric evaluation.
For the calculation of the dose estimate of the volunteers the MIRD formalism for an administration of a standard activity (37 kBq/1 mCi) of the radiolabeled pharmacon is applied. Animal biodistribution data are used to calculate the residence time in the source organs and tissues based on the maximum uptake f and biological half-life. In general a rat strain is used as animal model. As the organ weights in the rat and man are different an important correction of the animal data is necessary to estimate the f- values in humans. For each organ, dosimetric calculations are performed assuming (1) the same fraction of activity is absorbed by the organs in rat and humans irrespective of the difference in relative weight or (2) the fraction of activity absorbed by each organ is proportional to the relative organ weight in rat and humans. The latter assumption means that the uptake per kilogram of organ weight normalized to the whole body weight is the same for both species. Table 2 gives an overview of the organ and tissue weights in a male Wistar rat of 250 g reported in the literature (90) and in the standard human of 70 kg (32). For each organ or tissue two dose values are obtained by assuming a species independent organ uptake and an uptake proportional to the relative organ weight in different species. The highest dose estimate of both is restrained for each organ. If the retention for the individual organs is not known the whole body retention is adopted.
As model for the liver and biliary excretion it is generally assumed that a fraction of the radiopharmaceutical is taken up by the liver. Part of this activity goes directly to the small intestine while the resting part goes to the gallbladder, from where it is cleared to the small intestine. For the total fraction of activity excreted in this way by the gastrointestinal tract, the fraction of the activity retrieved in the feces is adopted from animal data. In general, data are available for different species and the maximal value is retained. For the dose calculation of the sections of the gastrointestinal tract, the kinetic model of the ICRP publication 53 is adopted (3). The kidney–bladder model described in this publication is also used to calculate the dose to the urinary bladder. Urine activity measurements in animals are used to estimate the fraction of the activity eliminated through the kidneys and again the maximal value is adopted if data are available for different species.
The dose estimates to organs and tissues of humans extrapolated in this way from animal data are combined

572 |
RADIOPHARMACEUTICAL DOSIMETRY |
|
|
|
|
Table 2. Organ Weights of a Male Wistar Rat of 250 ga and Human of 70 kgb |
|
||||
Organ |
|
RAT Weight, g |
RAT Rel. Weight, % |
Humans Weight, kg |
Humans Rel. Weight, % |
|
|
|
|
|
|
Adrenal glands |
0.085 |
0.034 |
0.014 |
0.020 |
|
Blood |
|
15 |
6 |
5.5 |
7.86 |
Bone |
|
12.99 |
5.19 |
5 |
7.14 |
Bone marrow |
5.59 |
2.24 |
3 |
4.29 |
|
Brain |
|
1.43 |
0.574 |
1.4 |
2.0 |
Heart |
|
0.835 |
0.334 |
0.33 |
0.47 |
Kidneys |
|
1.873 |
0.749 |
0.31 |
0.44 |
Large intestine |
2.635 |
1.054 |
0.37 |
0.53 |
|
Liver |
|
10.675 |
4.27 |
1.8 |
2.57 |
Lungs |
|
1.618 |
0.647 |
1 |
1.43 |
Oesophagus |
0.11 |
0.044 |
0.04 |
0.057 |
|
Pancreas |
|
0.913 |
0.365 |
0.1 |
0.14 |
Plasma |
|
10 |
4 |
3.1 |
4.43 |
Prostate |
|
0.3 |
0.12 |
0.016 |
0.023 |
Small intestine |
7.30 |
2.92 |
0.64 |
0.91 |
|
Spleen |
|
0.738 |
0.295 |
0.18 |
0.26 |
Stomach |
|
1.23 |
0.492 |
0.15 |
0.21 |
Testes |
|
1.815 |
0.726 |
0.035 |
0.050 |
Thymus |
|
0.593 |
0.237 |
0.02 |
0.029 |
Thyroid |
|
0.02 |
0.008 |
0.02 |
0.029 |
aSee Ref. 90.
bSee Ref. 32.
with the tissue weighting factors to obtain the effective dose after the administration of the standard activity (37 kBq/1 mCi) as described earlier (33). Based on the effective dose estimate obtained in this way and the dose limits proposed in the ICRP 62 publication (30) the activity of the radiolabeled drug to be administered to the volunteers is obtained.
CONCLUSIONS
To estimate the risk for late radiation effects as cancer and leukemia in patients after administration of radiopharmaceuticals for diagnosis the MIRD formalism with standard human anatomy and biokinetics is generally applied. This holds also for the estimation of the same risk of volunteers after the administration of a radiolabeled formulation of newly developed drugs. However, therapeutic applications of radiopharmaceuticals necessitate a reliable patient specific approach at least with respect to the biokinetics and if possible also for the patient-specific anatomical data. For curative treatment of malignant diseases there is now a tendency to use the largest safe dose approach with administration of the maximum possible activity based on the dose to the critical tissues. On the other hand, the advent of combined SPECT-CT or PET-CT imaging means an essential step forward toward an accurate 3D tumor dosimetry, the basic need for the administration protocols with the calculated activity based on a tumor dose prescription as used in external beam radiotherapy.
BIBLIOGRAPHY
1.Loevinger R, Berman M. A schema for absorbed-dose calculations for biologically distributed radionuclides. MIRD pamphlet No. 1. J Nucl Med 1968;9(Suppl.1):7–14.
2.Loevinger R, Budinger TF, Watson EE. MIRD primer for absorbed dose calculations, New York: Society of Nuclear Medicine; revised 1991.
3.ICRP publication 53. Radiation dose to patients from radiopharmaceuticals. Annals of the ICRP Vol 18. Oxford: Pergamon; 1987.
4.ICRP publication 80. Radiation dose to patients from radiopharmaceuticals. Annals of the ICRP Vol 28. Oxford: Pergamon press; 1998.
5.Stabin MG. MIRDOSE: personal computer software for internal dose assessment in nuclear medicine. J Nucl Med 1996;37:538–546.
6.Stabin MG, Sparks RB, Crowe E. OLINDA/EXM: The second generation personal computer software for internal dose assessment in nuclear medicine. J Nucl Med 2005;46:1023– 1027.
7.McDougall IR. Systemic radiation therapy with unsealed radionuclides. Sem Rad Oncol 2000;10:94–102.
8.Knox SJ, Meredith RF. Clinical radioimmunotherapy. Sem Rad Oncol 2000;10:73–93.
9.Robbins RJ, Schlumberger MJ. The evolving role of 131I for the treatment of differentiated thyroid carcinoma. J Nucl Med 2005;46:28S–37S.
10.Valde´s-Olmos RA, Hoefnagel CA. Radionuclide therapy in oncology: the dawning of its concomitant use with other modalities. Eur J Nucl Med Mol Imaging 2004;32:929–931.
11.Larson SM, Krenning EP. A pragmatic perspective on molecular targeted radionuclide therapy. J Nucl Med 2005; 46:1S–3S.
12.Kwekkeboom DJ, et al. Overview of results of peptide receptor radionuclide therapy with 3 radiolabeled somatostatin analogs. J Nucl Med 2005;46:62S–66S
13.Siegel JA, et al. MIRD Pamphlet No. 16: Techniques for quantitative radiopharmaceutical biodistribution data acquisition and analysis for use in human radiation dose estimates. J Nucl Med 1999;40:37S–61S.
14.Cristy M, Eckerman KF. Specific absorbed fractions of energy at various ages from internal photon sources. ORNL Report ORNL/TM-8381. Oak Ridge: Oak Ridge National Loboratory; 1987.
15.Giap HB, Macey DJ, Bayouth JE, Boyer AL. Validation of a dose-point kernel convolution technique for internal dosimetry. Phys Med Biol 1995;40:365–381.
16.Furhang EE, Sgouros G, Chui CS. Radionuclide photon dose kernels for internal emitter dosimetry. Med Phys 1996;23: 759–764.
17.Furhang EE, Chui CS, Sgouros G. A Monte Carlo approach to patient-specific dosimetry. Med Phys 1996;23:1523.
18.Liu A, Wiliams LE, Wong JYC, Raubitscek AA. Monte Carlo assisted voxel source kernal method (MAVSK) for internal dosimetry. J Nucl Med Biol 1998;25:423–433.
19.Yoriyaz H, Stabin MG, dos Santos A. Monte Carlo MCNP-4B- based absorbed dose distribution estimates for patient-specific dosimetry. J Nucl Med 2001;42:662.
20.Zaidi H, Sgouros G, editors. Therapeutic applications of Monte Carlo calculations in Nuclear Medicine. Bristol (UK): Institute of Physics Publishing; 2002.
21.Andreo A. Monte Carlo techniques in medical radiation physics. Phys Med Biol 1991;36:861–920.
22.Sgouros G, et al. Three-dimensional dosimetry for radioimmunotherapy treatment planning. J Nucl Med 1993;34:1595– 1601.
23.Guy MJ, Flux GG, Papavasileiou P, Flower MA, Ott RJ. RMDP-MC: a dedicated package for I-131 SPECT quantification, registration, patient-specific dosimetry and Monte Carlo. Seventh International Radiopharmaceutical Dosimetry Symposium. Proceedings of the International Symposium Nashville; (TN): Oak Ridge Associated Universities; 2002.
24.Gardin I. Voxeldose: a computer program for 3D dose calculation in therapeutic nuclear medicine. Seventh International Radiopharmaceutical Dosimetry Symposium. Proceedings of the International Symposium Nashville; (TN): Oak Ridge Associated Universities; 2002.
25.Kawrakow I, Rogers DWO. The EGSnrc code system: Monte Carlo simulation of electron and photon transport. NRC Report PIRS-701. Ottawa: National Research Council of Canada; 2000.
26.Carriers JF, Archembault L, Beaulieu L. Validation of GEANT4, an object-oriented Monte Carlo toolkit for simulations in medical physics.Med Phys 2004;31:484–492.
27.Weber S, Bauer A. Small animal PET: aspects of performance assessment. Eur J Nucl Med Mol Imaging 2004;31:1545–1555.
28.Sossi V, Ruth TJ. Micropet imaging: in vivo biochemistry in small animals. J Neural Transmission 2005;112:319–330.
29.Tai YC, et al. Performance evaluation of the microPET focus: a third generation microPET scanner dedicated to animal imaging. J Nucl Med 2005;46:455–463.
30.ICRP Publication 62. Radiological protection in biomedical research. Annals of the ICRP Vol. 22. Oxford: Pergamon; 1991.
31.Snyder WS, Ford MR, Warner GG, Watson SB. MIRD Pamphlet No. 11. ‘‘S’’, Absorbed dose per unit cumulated activity for selected radionuclides and organs. New York: Society of Nuclear Medicine; 1975.
32.ICRP Publication 23. Report of the task group on reference man. Oxford: Pergamon; 1975.
33.ICRP Publication 60. 1990 Recommendations of the International Commission on Radiological Protection. Oxford: Pergamon; 1991.
34.Piepsz A, et al. A radiopharmaceutical schedule for imaging in paediatrics. Eur J Nucl Med 1990;17:127–129.
35.Jacobs F, et al. Optimized tracer-dependent dosage cards to obtain weight-independent effective doses. Eur J Nucl Med Mol Imaging 2005;24:
36.Thierens H, Vral A, Van Haelst JP, Van de Wiele C, Schelstraete K, De Ridder L. Lymphocyte labeling with Technetium-99m-HMPAO: A Radiotoxicity Study using the Micronucleus Assay. J Nucl Med 1992;33:1167–1174.
RADIOPHARMACEUTICAL DOSIMETRY |
573 |
37.Goddu SM, et al. MIRD Cellular S values. New York: Society of Nuclear Medicine; 1997.
38.Bolch WE, et al. MIRD pamphlet No. 17: the dosimetry of nonuniform activity distributions-radionuclide S values at the voxel level. Medical Internal Radiation Dose Committee. J Nucl Med 1999;40:11S–36S.
39.Seo Y, et al. Correction of photon attenuation and collimator response for a body-contouring SPECT/CT imaging system. J NucL Med 2005;46:868–877.
40.Boucek JA, Turner JH. Validation of prospective whole-body bone marrow dosimetry by SPECT/CT multimodality imaging in I-131-anti-CD20 rituximab radioimmunotherapy of nonHodgkin’s lymphoma. Eur J Nucl Med Mol Imaging 2005;32: 458–469.
41.Coleman RE, et al. Concurrent PET/CT with an intergrated imaging system: Intersociety dialogue from the joint working group of the American College of Radiology, the Society of NuclearMedicine,andtheSocietyofComputedBodyTomography and Magnetic Resonance. J Nucl Med 2005;46:1225–1239.
42.Mawlawi O, et al. Performance characteristics of a newly developed PET/CT scanner using NEMA standards in 2D and 3D modes. J Nucl Med 2004;45:1734–1742.
43.Keidar Z, Israel O, Krausz Y. SPECT/CT in tumor imaging: technical aspects and clinical applications. Sem Nucl Med 2003;33:205–218.
44.Otte A, et al. Yttrium-90 DOTATOC: first clinical results. Eur J Nucl Med 1999;26:1439–1447.
45.Bodei L, et al. Receptor-mediated radionuclide therapy with 90Y-DOTATOC in association with amino acid infusion: a phase I study. Eur J Nucl Med Mol Imaging 2003;30:207–216.
46.Otte A, Cybulla M, Weiner SM. 90Y-DOTATOC and nephrotoxicity. Eur J Nucl Med Mol Imaging 2002;29:1543.
47.JamarF,etal.(86Y-DOTAA0)-D-Phe1-Tyr3-octreotide(SMT487): a phase I clinical study-pharmacokinetics, biodistribution and renal protective effect of different regimes of amino acid co-infusion. Eur J Nucl Med Mol Imaging 2003;30: 510–518.
48.Clarke SEM. Radionuclide therapy of the thyroid. Eur J Nucl Med 1991;18:984–991.
49.Howarth D, et al. Determination of the optimal minimum radioiodine dose in patients with Graves’disease: a clinical outcome study. Eur J Nucl Med 2001;28:1489–1495.
50.Guhlmann CA, Rendl J, Borner W. Radioiodine therapy of autonomously functioning thyroid nodules and Graves’disease. Nuklearmedizin 1995;34:20–23.
51.Willemsen UF, et al. Functional results of radioiodine therapy with a 300 Gy absorbed dose in Graves’disease. Eur J Nucl Med 1993;20:1051–1055.
52.Silver S. Radioactive nuclides in medicine and biology. Philadelphia: Lea & Febiger; 1968.
53.Coakley AJ, Thyroid stunning. Eur J Nucl Med 1998;25:203– 204.
54.van Isselt JW, et al. Comparison of methods for thyroid volume estimation in patients with Graves’disease. Eur J Nucl Med 2003;30:525–531.
55.Crawford DC, et al. Thyroid volume measurement in thyrotoxic patients: comparison between ultrasonography and iodine-124 positron emission tomography. Eur J Nucl Med 1997;24:1470–1478.
56.Bockisch A, Jamitzky T, Derwanz R, Biersack HJ. Optimized dose planning of radioiodine therapy of benign thyroidal diseases. J Nucl Med 1993;34:1632–1638.
57.Kalinyak JE, McDougall IR. Editorial: How should the dose of iodine-131 be determined in the treatment of Graves’ hyperthyroidism ? J Clin Endocrinol Metab 2003;88:975–977.
58.Leslie WD, et al. A randomized comparison of radioiodine doses in Graves’hyperthyroidism. J Clin Endocrinol Metab 2003;88:978–983.