
Environmental Biotechnology - Jordening and Winter
.pdf
304 11 Bioreactors
Another example of composting contaminated soil (in a column) was presented by Gorostiza et al. (1998). In a soil column the degradation of pentachlorophenol with and without added compost was tested; compost addition significantly enhanced the degradation rate (residence time about 60 d).
On a larger scale (in Finland), composting systems have been implemented by mixing contaminated soil with spruce bark chips as a bulking agent, lime, and nutrients beforehand. The bed is constructed as a biopile and can be turned by a trac- tor-drawn screw mixer. Degradation temperature rose during composting to as high as 44 °C. During five months the mineral oil concentration dropped from 2400 to 700 mg kg–1 (Puustinen et al., 1995).
In another experiment, four biopiles of 10 m3 each were created to treat chloro- phenol-contaminated soil. Chalk, commercial fertilizer (NPK), and bark chips (as a bulk aeration agent) were added. After two months 80% of the contaminant was removed (Laine and Jorgensen, 1995).
11.4.3
(Semi)Continuous Operation: The Rotating-drum Bioreactor
In a rotating-drum bioreactor (or revolving tubular reactor), the reactor itself acts as a rotating conveyer in which the contaminated solids are mixed (Fig. 11.3). In a (semi)continuous operation the solids are transported (screwed) through the system. Loading at the head of the process is combined with unloading at the back. The solids residence time in the system is determined by the shape of the screw blades, the rotation speed, the scale, and the amount of material present. Experiments have been carried out in a bioreactor with a length of 25 m and a diameter of 3.5 m. A warm air blower provided an appropriate temperature and oxygen, a sprinkler system was installed and nutrients added; the residence time was 14 d. For starting concentrations of mineral oil contaminant ranging from 1000 to 6000 mg kg–1, at a temperature of 22 °C, the contaminant concentration decreased to 50–350 mg kg–1. Most breakdown activity took place in the first few days (Munckhof and Veul, 1990).
In a revolving tubular reactor developed by BioteCon in Germany, having a length of 18 m and a diameter of 2.5 m, between 0.5 and 1 t h–1 of soil can be treated (Kiehne et al., 1995). Model drums with diameters of 0.5 m and 1 m were tested. The typical moisture content was 15%, the revolution speed was 1 h–1, and the aeration rate 0.125 VVM. In the trials the breakdown of motor fuel, kerosene, and phenol was investigated. Under batch conditions kerosene was degraded from 8300 mg kg–1 to 500 mg kg–1 in 300 h. When the same soil was treated in a repeated batch (25% of the treated soil was returned and mixed with new soil), within 180 h end concentrations of 180 mg kg–1 were reached. The increase in microbial activity due to the reuse of biomass was considered responsible for the enhanced breakdown.
In an experiment a combination between heap leaching and reactor processing was tested. After treatment in the reactor for 120 h, kerosene-contaminated soil was deposited in a heap for four weeks. The treatment was finalized by a second reactor treatment for 100 h. With a total residence time in the reactor of 220 h, an overall conversion of 98.5% was achieved. It was concluded that for “contaminated soils in
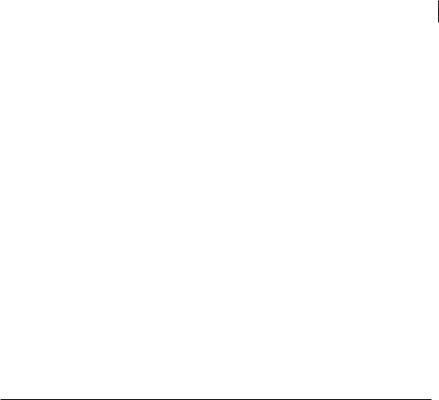
11.5 Comparison of Bioreactors 305
the order of 500–3000 t the revolving tubular reactor especially demonstrates its advantage” (Kiehne et al., 1995). The technology has been developed to operate on-site.
Another solid-state process was developed by Umweltschutz–Nord (Bremen, Germany). Using a steel tube having a length of 45 m and an diameter of 3 m, the contaminated soil is screwed through the Terranox system. For mineral oil-contaminat- ed soils a treatment period of 6–8 weeks is used; the system can also be used for a combination of anaerobic and aerobic treatments.
On a smaller scale (3, 6, and 90 L) a blade mixing bioreactor was operated as a sol- id-state process (Hupe et al., 1995). The authors concluded that, in addition to the benefit of adding compost, moisture enhances the process; however, the water content should be <65% of the maximum moisture content to obtain solid-state processing conditions. The aeration rate is also a key parameter.
11.5
Comparison of Bioreactors
Table 11.1 shows a technological and economical characterization of the three bioreactor systems. It is clear that composting is the ‘low technology’ type of bioreactor, requiring only limited technological infrastructure and investment. Slurry process-
Table 11.1 Comparison of bioreactors.
Technology |
Economics |
||
|
|
||
|
Biological slurry processing |
||
• |
Batch and (semi)continuous |
• |
Substantial investment (equipment) |
• |
Water addition |
• Complex plant or trained operators required |
|
• |
Energy input |
• Large scale needed (economy of scale) |
|
• Addition of nutrients and/or biomass |
• Use as recycling technology |
||
• Controlled conditions in closed systems |
• Cost per ton determined by surface, |
||
|
|
|
machinery, energy, and labor costs |
|
Composting |
||
• |
Batch operation |
• |
Low investment |
• Limited use of technology and machinery |
• |
Large surface required |
|
• |
Low energy input |
• Use as decontamination technology only |
|
• Addition of compost to the solids |
• May be used on small scale |
||
• |
Limited control options |
• Cost per ton determined by surface and |
|
• |
Longer treatment times |
|
labor costs |
|
Rotating solids bioreactor |
||
• |
(Semi)continuous |
• |
Substantial investment (equipment) |
• |
Limited control options |
• |
Mobile plant on-site |
• |
Moderate scale required |
• |
Small volumes |
• |
Moderate energy input |
• Cost per ton similar to that of slurry systems |
|
|
|
|
|

306 11 Bioreactors
ing requires a technological infrastructure, and rotating-drum systems have an intermediate position.
Experiments show that during batch processing, the microbial breakdown slows down at low concentrations and full conversion is not reached. Continuous processing significantly improves the results.
The economic feasibility of bioprocessing depends largely on the nature of the waste streams to be treated. Basically, waste streams having a large water content and a considerable fine fraction (which is predominantly contaminated with organic components) are suitable for bioprocessing.
From the economic point of view, continuous slurry processing in an off-site installation can be beneficial only on a large scale. Capacities >40 000–60 000 t a–1 (depending on the local conditions) are needed to benefit from the economy of scale and thus to perform at acceptable cost levels. For batch-operated composting processes, smaller volumes, handled close to or on the site, may offer solutions if sufficient area is available and time is not limited. Rotating-drum systems in which sol- id-state processing is achieved are considered to perform well for smaller volumes (up to 3000 t) in a mobile plant used on-site.
11.6
Conclusions and Outlook
11.6.1
Conclusions
•In terms of product quality, energy consumption, and costs, bioprocessing has the capability to function as a useful waste recycling technology.
•Comparing the three bioreactor types considered, we can conclude that
–slurry processing offers the best option for rapid, controlled integral treatment
–composting can be used as a batch approach (biopiles) for smaller amounts
–rotating-drum bioreactors are a solid-state alternative to slurry processing
•In the decontamination market, bioreactor processing should focus on wet waste streams having a large content of contaminated fines; the contamination should be essentially organic in character.
•Unambiguous analytical techniques and procedures and clear (international) recycling standards are needed to properly evaluate the various technologies, the end products, and calibration standards.
•Research has to identify the reasons for the hampered breakdown as measured in various field experiments. Especially for large input concentrations, the bioavailability (or even toxicity) of the contaminants may seriously interfere with optimal microbial breakdown conditions. Ways have to be found to overcome these limitations.
•To fully explore the possibilities of bioprocessing, notice should be taken of the benefits of (semi)continuous processing.

References 307
11.6.2
Outlook
•The trend toward increasing use of environmental biotechnology will result in the availability of organisms able to degrade almost any organic contaminant. To transform the potentials of these organisms into feasible bioprocesses, the proper combination of bioreactors and separation techniques (to remove the clean fractions such as sand) is crucial.
•Various options focusing on the integration of bioreactors with landfarming, ripening, or phytoremediation will be explored. In addition, in situ treatment based on bioreactor research, such as biorestoration or bioscreens will be fully developed.
•Biotechnological processes will be explored and used on a larger scale. Owners of contaminated sites that might be treated by bioprocesses have to benefit from legal and financial stimuli such as eco taxes on waste disposal.
References
Apitz, S. E., Pickwell, G. V., Meyer-Schulte, K. J., Kirtay, V., Douglass, E., A slurry biocascade for the enhanced degradation of fuels in soils, Fed. Environ Restoration and Waste Minimization Conf. Vol. 2, New Orleans 1994, pp. 1288– 1299.
Balen van, A., Influence of the water activity on Zymomonas mobils, Thesis, Technical University of Delft, The Netherlands 1991.
Blank-Huber, M., Huber, E., Huber, S., Hutter, J., Heiss, R., Development of a mobile plant, in: Proc. Soil Decontamination Using Biological Processes, Karlsruhe. Frankfurt/ Main 1992: Dechema.
Brady, N. C., Nature and Properties of Soils.
New York 1984: Macmillan. Brummelen ten, E., Oostra, R., Pruijn, M.,
Weller, B., Bioremediation with the Fortec process: a safe harbour for sediment, Int. Conf. Contaminated Sediments, preprints Vol. 1, p. 405, Rotterdam, The Netherlands
1997.
Building Material Act, Bouwstoffenbesluit boden: en oppervlaktewaterbescherming, TK 22683, Staatsdrukkerij, Den Haag, The Netherlands 1995.
Crank, J., The Mathematics of Diffusion, 2nd edn. Oxford 1975: Clarendon.
Cullinane, M. J., Averett, D. E., Shafer, R. A., Male, J. M., Truitt, C. L., Bradbury, M. R.,
Contaminated Dredged Material, New Jersey
1990: Noyes Data Corporation.
Gorostiza, I., Susaeta, I., Bibao, V., Diaz, A. I., San Vicente, A. I., Salas, O., Biological removal of wood preservative (PCP) waste in soil, autochtonous microflora and effect of compost addition, in: Proc. Contaminated Soil, p. 1149. London 1998: Thomas Telford.
Harmsen, J., in: Possibilities and Limitations of Landfarming, On-Site Bioreclamation (Hincheeve, Olfenbuttel, eds.). London 1991: Butterworth Heinemann.
Hinze, J. O., Turbulence. New York 1959: McGraw-Hill.
Hupe, K., Heerenklage, J., Luth, J., Stegmann, R., Enhancement of the biological degradation processes in contaminated soils, in: Proc. Contaminated Soil (Van de Brink, W. J., Bosman, R., Arendt, F., eds.), p. 853. Dordrecht 1995: Kluwer.
INES, Project Bioslib, Stichting Europort Botlek Belangen, The Netherlands 1997.
Jerger, D., Cady, D. J., Bentjen, S., Exner, J., Full scale bioslurry reactor treatment of creo- sote-contaminated material at south eastern wood preserving Superfund site, in: Speaker Abstract of In-situ and On-site Bioreclamation, 2nd Int. Symp. San Diego, CA 1993.
Kiehne, M., Berghof, K., Muller-Kuhrt, L., Buchholz, R., Mobile revolving tubular reactor for continuous microbial soil decontamination, in: Proc. Contaminated Soil (Van de Brink, W. J., Bosman, R., Arendt, V., eds.), p. 873. Dordrecht 1995: Kluwer.

30811 Bioreactors
Kleijntjens, R. H., Biotechnological slurry process for the decontamination of excavated polluted soils, Thesis, Technical University of Delft, The Netherlands 1991.
Kleijntjens, R. H., Kerkhof, L., Schutter, A. J., Luyben, K. C. A. M., The slurry decontamination process, bioprocessing of contaminated solid waste streams, Abstract 2489,
Abstract Book of the 9th European Congress of Biotechnology, July 1999, Brussels.
Koning, M., Hupe, K., Luth, C., Cohrs, C., Stegmann, R., Comparative investigation into biological degradation in fixed bed and slurry reactors, in: Proc. Contaminated Soil, p. 531. London 1998: Thomas Telford.
Laine, M., Jorgensen, S., Pilot scale composting of chlorophenol-contaminated saw mill soil, in: Proc. Contaminated Soil (Van de Brink, W. J., Bosman, R., Arendt, F., eds.), p. 1273. Dordrecht 1995: Kluwer.
Levenspiel, O., Chemical Reaction Engineering. New York 1972: Wiley.
Lotter, S., Stregmann, R., Heerenklage, J., Basic investigation on the optimization of biological treatment of oil contaminated soils, in: Proc. Contaminated Soil (Van de Brink, W. J., Bosman, R., Arendt, F., eds.), p. 967. Dordrecht 1990: Kluwer.
Luyben, K., Kleijntjens, R., Werkwijze en inrichting voor het scheiden van vaste stoffen, Dutch Patent 8802728, Den Haag 1988.
Munckhof, P., Veul, F., Production scale trials on the decontamination of oil polluted soil in a rotating bioreactor at field capacity, in: Proc. Contaminated Soil (Van de Brink, W. J., Bosman, R., Arendt, F., eds.). Dordrecht 1990: Kluwer.
Nitschke, V., Entwicklung eines Verfahrens zur mikrobiologischen Reinigung feinkörniger mit PAH belasteter Boden, Thesis, Universität Gesamthochschule Paderborn, Germany
1994.
Northcliff, S., Bannick, C., Paetz, A., International standardization for soil quality, in:
Proc. Contaminated Soil, May 1998, Edinburgh, pp. 83–91. London 1998: Thomas Telford.
Oostenbrink, I., Kleijntjens, R., Mijnbeek, G., Kerkhof, L., Vetter, P., Luben, K., Biotechnological decontamination using a 4 m3 pilot plant of the slurry decontamination process, in: Proc. Contaminated Soil (Van de Brink, W. J., Bosman, R., Arendt, F., eds.). Dordrecht1995: Kluwer.
Puustinen, K., Jorgensen, S., Strandberg, T., Suortti, M., Bioremediation of oil contaminated soil from service stations: evaluations of biological treatment, in: Proc. Contaminated Soil (Van de Brink, W. J., Bosman, R., Arendt, F., eds.), p. 1325. Dordrecht 1995: Kluwer.
Reynaarts, H., Bachmann, A., Jumelet, J., Zehnder, A., Effect of desorption and mass transfer on the aerobic mineralization of α- HCH in contaminated soils, Environ. Sci. Technol. 1990, 24, 1493.
Riser-Roberts, E., Remediation of Petroleum Contaminated Soils. Boca Raton, FL 1998: CRC Press.
RIZA Report 97-067, Hoofdrapport pilot sanering Petroleumhaven Amsterdam: monitoring en evaluatie. SDU-Den Haag, The Netherlands
1997.
Robra, K., Somitsch, W., Becker, J., Jernej, J., Schneider, M., Battisti, A., Off-site bioremediation of contaminated soil and direct reutilization of all oil fractions, in: Proc. Contaminated Soil. London 1998: Thomas Telford.
Schaeffer, G., Hattwig, S., Unterste, M., Hupe, K., Heerenklage, J. et al., PAH degradation in soil: microbial activity or inoculation, in:
Proc. Contaminated Soil (Van de Brink, W. J., Bosman, R., Arendt, F., eds.), p. 415. Dordrecht 1995: Kluwer.
Schlegel, H. G., Allgemeine Mikrobiologie. Stuttgart 1986: Thieme.
Sinder, C., Klein, J., Pfeifer, F., The DMT–Bio- dyn process, a suspension reactor for biological treatment of fine grained soil contaminated with PAH, Abstract 2812, Abstract Book of the 9th European Congress of Biotechnology, July 1999, Brussels 1999.
Suzuki, M., Waste management according to Japanese experience, 4th World Congr. Chemical Engineering, June 1991, Dechema, Frankfurt, Germany 1992.
Teschner, M., Wehner, H., Chromatographic investigations on biodegraded crude oils,
Chromatographia 1985, 2, 407–416.
Toro, Di D. M., Horzempa, L., Reversible and resistant components of PCB adsorption–de- sorption isotherms, Environ. Sci. Technol.
1982, 16, 594–602.
Warbout, J., Ouboter, P. S. H., Een vergelijking tussen verschillende methode om het gehalte aan minerale olie in grond te bepalen, H2O
1988, 21, 1.

Werther, J., Wilichowski, M., Investigations in the physical mechanisms involved in washing processes, in: Proc. Contaminated Soil (Arendt, F., Hinsenveld, M., Van de Brink, W. J., eds.), pp. 907–920. Dordrecht 1990: Kluwer.
References 309
Wu, S., Geschwend, P., Numerical modelling of sorption kinetics of organic compounds to soil and sediment particles, Water Res. Des. 1988, 24, 1373–1383.

311
12
In-situ Remediation
T. Held and H. Dörr
12.1 Introduction
The input of contaminants into soil and groundwater may lead to a persistent pollution. The methods for remediation of the environmental compartments contaminated with organic contaminants comprise, in addition to physical and chemical methods, also biological technologies. The technologies are subdivided into ex situ and in situ methods. Ex situ methods include excavation of the soil and subsequent treatment at the site (on-site) or elsewhere (off-site). In situ means that the soil remains in its natural condition during treatment. Generally, in situ technologies also include ex situ components, e.g., wateror gas-treatment plants.
The goal of in situ technologies is to mineralize the contaminants microbiologically to form harmless end products. Many biodegradation processes, especially of nonchlorinated compounds (e.g., mineral oil hydrocarbons), require aerobic conditions or denitrification. Although these contaminants may also be degraded under other conditions (e.g., iron reduction, sulfate reduction), these bioprocesses are not very effective and are therefore not used in enhanced bioremediation. These processes are productive, i.e., the contaminants serve as sources of carbon and energy. Chlorinated hydrocarbons usually require anaerobic conditions. They may be degraded productively (during dehalorespiration, chlorinated hydrocarbons serve as obligate electron acceptors) or co-metabolically. In the latter, microorganisms cannot grow on these contaminants but need an additional substrate for growth and biodegradation. Additional nutrient elements such as nitrogen and phosphate as well as electron acceptors are often lacking. In addition to such mineralization, other biochemical processes that result in a reduction in toxicity can also be applied in in situ remediation processes. These are
•cometabolic aerobic or anaerobic transformation
•humification
•precipitation
•solubilization
•volatilization
Environmental Biotechnology. Concepts and Applications. Edited by H.-J. Jördening and J. Winter Copyright © 2005 WILEY-VCH Verlag GmbH & Co. KGaA, Weinheim
ISBN: 3-527-30585-8
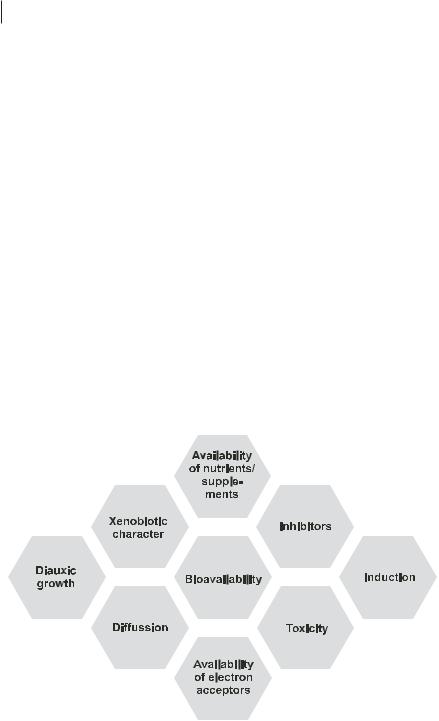
312 12 In-situ Remediation
With the use of these processes, a wide variety of contaminants seem to be treatable in situ, including
•mineral oil hydrocarbons
•monoaromatic and polyaromatic compounds
•chlorinated or nitrated aliphatics and aromatics
•inorganic ions, including simple and complex cyanides
•heavy metals
However, until now only a limited number of possible techniques have been developed for practical application. The main reason for the actual state of development is that the in situ degradability of contaminants is limited by numerous factors (Fig. 12.1). Most of these factors, like low solubility, strong sorption on solids, sequestration with high molecular weight matrices, diffusion into macropores of soils and sediments, scavenging by insoluble and lipophilic phases, lead to a limited mass transfer. This lack of bioavailability may prevent sufficient degradation and result in persistence of the contaminants.
While planning an in situ remediation process, one has to consider that the technology covers processes working on various scales (from nanometers to kilometers) (Fig. 12.2). Import and export of contaminants by bacterial cells and induction of the degradative enzymes occurs on the nanometer scale. Surface processes occur on the micrometer scale. In the range of micrometers to millimeters diffusion processes (soil micro pores) as well as micro-inhomogeneities occur. The meter scale represents small inhomogeneities, e.g., silt lenses in a sandy aquifer. Finally, the kilome-
Fig. 12.1 Limiting factors influencing the degradability of contaminants.
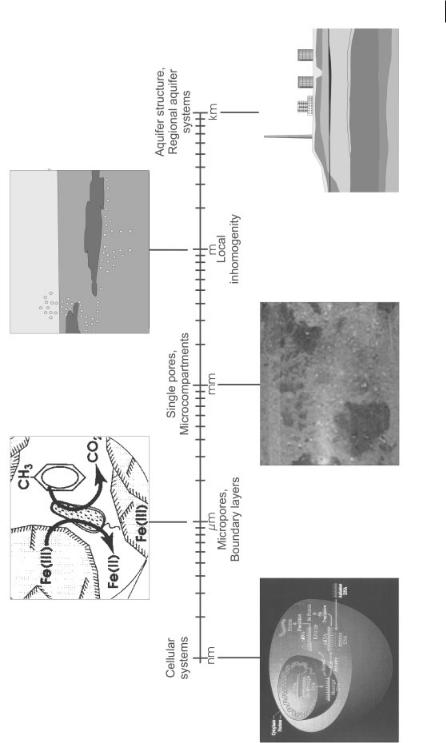
12.1 Introduction 313
Processes on different scales.
Fig. 12.2

314 12 In-situ Remediation
ter scale covers local aquifer systems. From scale to scale, different constraints limit the processes. In general, the constraints of the largest scale, i.e., hydraulic site conditions, might be the overall limiting processes. Hydraulically favorable conditions are offered by course sediments with a hydraulic conductivity of >10–4 m s–1.
Some of the main features of in situ technologies are to transport supplements (nutrient salts, electron acceptors or donors) to the contaminants and to remove reaction products, as well as to induce proper environmental conditions for contaminant degradation. This may, e.g., be implemented by pumping of reaction-pro- duct–enriched groundwater, water cleaning, and reinfiltration of water enriched with nutrients.
The efficiency of in situ biodegradation depends importantly on the distribution of the nutrients in suitable concentrations within the aquifer. Biodegradation processes that require aerobic conditions may be limited by the lack of oxygen. The maximum O2 concentration dissolved in water that can be infiltrated is given by its solubility of about 50 mg L–1. The oxygen supply can be increased by using hydrogen peroxide (H2O2), which is decomposed to O2 and water enzymatically or by geogenic components. However, the concentrations of H2O2 in the infiltration water are limited by its toxicity to bacteria at concentrations above 1000 mg L–1 and the possibility of O2 bubble formation, which would lead to a decrease in hydraulic conductivity and thus to decreased nutrient and oxygen supply.
The demand for oxygen or other electron acceptors decreases during the course of the remediation. In the beginning, electron acceptor consumption is usually limited by the supply rate. In a later phase, when all readily available compounds have been degraded, the electron acceptor consumption is limited by the rate of contaminant dissolution or diffusion out of micropores. Especially in this phase is it important, if O2 is added, to use proper O2 infiltration concentrations to avoid bubble formation.
The use of denitrifying conditions for contaminant degradation by the infiltration of nitrate may also lead to the formation of bubbles, because the end product of the denitrification is nitrogen (N2), which has a comparably low solubility. If nitrate is overdosed or the exfiltration rate is too slow, this may result in N2 degassing and bubble formation.
12.2 Investigations
Each site has to be investigated not only for its geological, hydrogeological, and contaminant situation, but also for the site-specific degradability. The following description refers to laboratory methods for investigation of the microbiological degradability of contaminants. These methods are divided into two stages [1] (Fig. 12.3). During the first stage the general degradability is determined, i.e., whether the contaminants at the given site are sufficiently degradable. Conclusive demonstration of the fate of the contaminants can be obtained only by using tracer (e.g., 14C-labeled substances). However, this is possible only in closed laboratory systems and not on a