
Environmental Biotechnology - Jordening and Winter
.pdf
References 201
tance in the production of gases which have an inhibitory effect on methane generation like H2S and NH3.
The influence of hydrostatic pressure on biogas production (Section 6.6) should be taken into consideration for most large technical scale bioreactors with a height of more than 5 m.
List of Symbols
A |
area |
m2 |
|
|
|
a |
interfacial area per volume |
m2 |
m–3 |
||
ci |
concentration of component i |
mol L–1 |
|||
D |
diffusion coefficient |
m2 |
s |
|
|
db |
diameter, bubble |
m |
|
|
|
G |
gas volume |
m3 |
|
|
|
Hi |
Henry coefficient of component i |
mol L–1 Pa–1 |
|||
Ki |
kinetic constant of component i |
mol L–1 |
|||
KD,i |
dissociation coefficient of component i |
mol L–1 |
|||
k |
proportional coefficient |
h–1 |
|
|
|
kL a |
volume specific mass transport coefficient |
h–1 |
|
|
|
· |
produced biomass |
|
|
–1 |
|
Mprod |
g h |
|
|||
· |
sedimenting mass flow from module i |
|
|
–1 |
|
Msedi,i |
g h |
|
|||
pi |
partial pressure of component i |
Pa |
|
|
|
R |
universal gas constant, R = 8.314 kJ kg–1 K–1 |
kJ kg–1 K–1 |
|||
SSi |
concentration of suspended solids in module i |
g L–1 |
|
||
T |
absolute temperature |
K |
|
|
|
TOC |
total organic carbon |
g L–1 |
|
||
u |
velocity |
m s–1 |
|||
w |
velocity |
m s–1 |
|||
V |
volume |
m3 |
|
|
|
· |
exchange flow rate |
m |
3 |
h |
–1 |
Vexchange |
|
|
|||
· |
volumetric gas production rate |
m |
3 |
h |
–1 |
Vbubble |
|
|
|||
· |
feed flow rate |
m |
3 |
h |
–1 |
Vfeed |
|
|
|||
· |
gas flow rate |
m |
3 |
h |
–1 |
Vgas |
|
|
|||
· |
recycling gas flow rate |
m |
3 |
h |
–1 |
Vrecyc. |
|
|
|||
x |
concentration of biomass |
g L–1 |
|
||
Y |
yield coefficient |
g mol–1 |
Greek
ãi |
activity coefficient [–] |
åG |
gas hold up [–] |
Äå |
difference of gas hold up [–] |
ô |
boundary layer renewal time s |
Special Symbols of Chemical Components
Pro |
total propionic acid |
HPro |
undissociated propionic acid |
Pro– |
dissociated propionic acid |

2026 Modeling of Biogas Reactors
References
Aivasidis, A., Bastin, K., Wandrey, C., Anaerobic Digestion 1981, p. 361. Amsterdam 1982: Elsevier.
Beutier, D., Renon, H., Representation of
NH3UH2SUH2O, NH3UCO2UH2O and NH3USO2UH2O vapor–liquid equilibria,
Ind. Eng. Chem. Process Des. 1978, 17, 220–230.
Blenke, H., Biochemical loop reactors, in: Bi- otechnology 1st Edn., Vol. 2, Fundamentals of Biochemical Engineering, pp. 465–517. Weinheim 1985: VCH.
Friedmann, H., Märkl, H., Der Einfluß von erhöhtem hydrostatischen Druck auf die Biogasproduktion, Wasser-Abwasser gwf
1993, 134, 689–698.
Friedmann, H., Märkl, H., Der Einfluß der Produktgase auf die mikrobiologische Methanbildung, Wasser-Abwasser gwf 1994, 6, 302–311.
Fukuzaki, S., Nishio, N., Nagai, S., Kinetics of the methanogenic fermentation of acetate, Appl. Environ. Microbiol. 1990, 56, 3158–3163.
Graef, S. P., Andrews, J. F., Mathematical modeling and control of anaerobic digestion, AIChE Symp. Series 1973, 70, 101–131.
Huser, B. A., Wuhrmann, K., Zehnder, A. J. B., Methanothrix soehngenii gen. nov., a new acetotrophic non-hydrogen oxidizing methane bacterium, Arch. Microbiol. 1982, 132, 1–9.
Loewenthal, R. E., Marais, G. v. R., Carbonate Chemistry of Aquatic Systems: Theory and Application, Vol. 1. Ann Arbor, MI 1976: Science Publishers.
Märkl, H., Reinhold, G., Biogas-Turmreak- tor, ein neues Konzept in der anaeroben Abwasserreinigung, Chem.-Ing. Tech. 1994, 66, 534–536.
Märkl, H., Mather, M., Witty, W., Meßund Regeltechnik bei der anaeroben Abwasserreinigung sowie bei Biogasprozessen.
Münchener Beiträge zur Abwasser-, Fischereiund Flußbiologie, Vol. 36, pp. 49–64. München, Wien 1983: R. Oldenbourg Verlag.
Matz, G., Lennemann, F., On-line monitoring of biotechnological processes by gas chromatographic mass-spectrometric analysis of fermentation suspensions, J. Chromatogr. A 1996, 750, 141– 149.
Meyer-Jens, T., Matz, G., Märkl, H., Online measurement of dissolved and gaseous hydrogen sulphide in anaerobic biogas reactors,
Appl. Microbiol. Biotechnol. 1995, 43, 341– 345.
Morvai, L., Miháltz, P., Holló, J., Comparison of the kinetics of acetate biomethanation by raw and granular sludges, Appl. Microb. Biotechnol. 1992, 36, 561–567.
Polomski, A., Einfluß des Stofftransports auf die Methanbildung in Biogas-Reaktoren. Thesis, Technical University Hamburg-Har- burg, Germany (1998).
Reinhold, G., Märkl, H., Model-based scale-up and performance of the Biogas Tower Reactor for anaerobic waste water treatment, Wa- ter Res. 1997, 31, 2057–2065.
Reinhold, G., Merrath, S., Lennemann, F., Märkl, H., Modeling the hydrodynamics and the fluid mixing behavior of a Biogas Tower Reactor, Chem. Eng. Sci. 1996, 51, 4065–4073.
Thauer, R. K., Jungermann, K., Decker, K., Energy conservation in chemotrophic anaerobic bacteria, Bact. Rev. 1977, 41, 100–180.
Therkelsen, H. H., Carlson, D. A., Thermophilic anaerobic digestion of a strong complex substrate, J. Water Pollut. Control. Fed.
1979, 51, 7, 1949–1964.
Therkelsen, H. H., Sörensen, J. E., Nielsen, A. M., Thermophilic Anaerobic Digestion of Wastewater Sludge and Farm Manure, Project Brief of COWI-Consult in 45 Teknikerbyen, DK2830 Virum (1981).
Weiland, P., Untersuchungen eines Airliftreaktors mit äußerem Umlauf im Hinblick auf seine Anwendung als Bioreaktor. Thesis, University of Dortmund, Germany (1978).
Witty, W., Märkl, H., Process engineering aspects of methanogenic fermentation on the example of fermentation of Penicillium mycelium, Ger. Chem. Eng. 1986, 9, 238–245.
Yang, S. T., Okos, M. R., Kinetic study and mathematical modeling of methanogenesis of acetate using pure cultures of methanogens, Biotechnol. Bioeng. 1987, 30, 661–667.
Zumbusch, P. von, Meyer-Jens, T., Brunner, G., Märkl, H., On-line monitoring of organic substances with high-pressure liquid chromatography (HPLC) during the anaerobic fermentation of waste water, Appl. Microbiol. Biotechnol. 1994, 42, 140–146.

203
7
Aerobic Degradation of Recalcitrant Organic Compounds by Microorganisms
Wolfgang Fritsche and Martin Hofrichter
7.1
Introduction: Characteristics of Aerobic Microorganisms Capable of Degrading Organic Pollutants
The most important classes of organic pollutants in the environment are mineral oil constituents and halogenated products of petrochemicals. The capacities of aerobic microorganisms are of particular relevance for the biodegradation of such compounds and are described as examples with reference to the degradation of aliphatic and aromatic hydrocarbons as well as their chlorinated derivatives. The most rapid and complete degradation of the majority of pollutants is brought about under aerobic conditions.
The following are essential characteristics of aerobic microorganisms degrading organic pollutants (Fig. 7.1):
•Metabolic processes for optimizing the contact between the microbial cells and the organic pollutants. The chemicals must be accessible to the organisms having biodegrading activities. For example, hydrocarbons are water-insoluble and their degradation requires the production of biosurfactants.
•The initial intracellular attack on organic pollutants is an oxidative process; the activation and incorporation of oxygen is the enzymatic key reaction catalyzed by oxygenases and peroxidases.
•Peripheral degradation pathways convert the organic pollutants step by step into intermediates of the central intermediary metabolism, e.g., the tricarboxylic acid cycle.
•Biosynthesis of cell biomass from the central precursor metabolites, e.g., acetylCoA, succinate, pyruvate. Sugars required for various biosyntheses and growth must be synthesized by gluconeogenesis.
A huge number of bacterial and fungal genera possess the ability to degrade organic pollutants. Biodegradation is defined as the biologically catalyzed reduction in complexity of chemical compounds (Alexander 1994). It is based on two processes: growth and cometabolism. In growth, an organic pollutant is used as sole source of
Environmental Biotechnology. Concepts and Applications. Edited by H.-J. Jördening and J. Winter Copyright © 2005 WILEY-VCH Verlag GmbH & Co. KGaA, Weinheim
ISBN: 3-527-30585-8
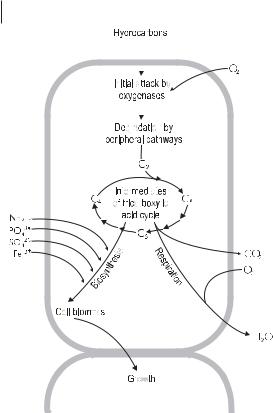
204 7 Aerobic Degradation of Recalcitrant Organic Compounds by Microorganisms
Fig. 7.1 Main characteristics of aerobic degradation of hydrocarbons: processes associated with growth of microorganisms.
carbon and energy. This process results in a complete degradation (mineralization) of organic pollutants, as demonstrated in Section 7.2.2. Cometabolism is defined as the metabolism of an organic compound in the presence of a growth substrate that is used as the primary carbon and energy source. The principle is explained in Section 7.2.4.
Key enzymatic reactions of aerobic biodegradation are oxidations catalyzed by oxygenases and peroxidases. Oxygenases are oxidoreductases that use O2 to incorporate oxygen into the substrate. Degradative organisms need oxygen at two metabolic sites
– the initial attack on the substrate and the end of the respiratory chain (Fig. 7.1). Certain higher fungi have developed a unique oxidative system for the degradation of lignin based on extracellular ligninolytic peroxidases and laccases. This enzymatic system possesses increasing significance for the cometabolic degradation of persistent organopollutants. Thus, the basidiomycetous fungi require deeper insights and extensive consideration. Therefore, this chapter is divided into two sections: bacterial and fungal degradation.
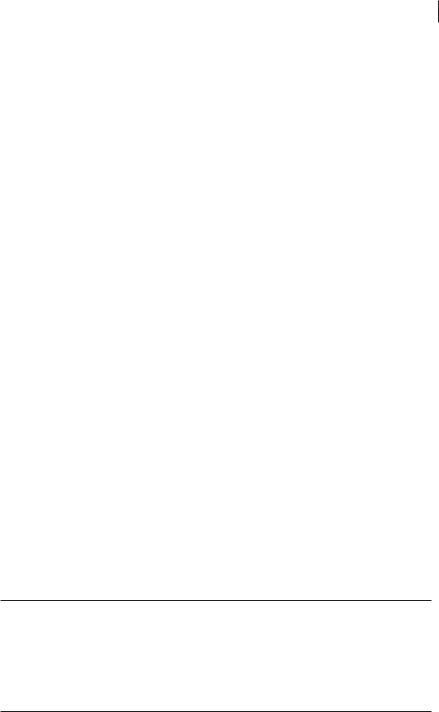
7.2 Principles of Bacterial Degradation 205
7.2
Principles of Bacterial Degradation
7.2.1
Typical Aerobic Degrading Bacteria
The predominant degraders of organopollutants in the oxic zone of contaminated areas are chemo-organotrophic species, which are able to use a huge number of natural and xenobiotic compounds as carbon sources and as electron donors for the generation of energy. Although many bacteria are able to metabolize organic pollutants, a single bacterial species does not possess the enzymatic capability to degrade all or even most of the organic compounds in a polluted soil. Mixed microbial communities have the most powerful biodegradative potential, because the genetic information of more than one organism is necessary to degrade the complex mixtures of organic compounds present in contaminated areas. The genetic potential and certain environmental factors such as temperature, pH, and available nitrogen and phosphorous sources, therefore, seem to determine the rate and the extend of degradation.
The predominant bacteria in polluted soils belong to a spectrum of genera and species (Table 7.1). The table lists only bacteria that can be cultured on nutrient-rich media. We have to consider that the majority of bacteria present in soils cannot yet be cultivated in the laboratory.
The pseudomonads, aerobic gram-negative rods that never show fermentative activity, seem to have the highest degradative potential, e.g., Pseudomonas putida and P. fluorescens. Further important degraders of organic pollutants are found within the genera Comamonas, Burkholderia, and Xanthomonas. Some species utilize >100 different organic compounds as carbon sources. The immense potential of the pseudomonads does not solely depend on the catabolic enzymes, but also on their capability for metabolic regulation (Houghton and Shanley 1994). A second important group of degrading bacteria are the gram-positive rhodococci and coryneform bacteria. Many species, now classified as Rhodococcus spp., were originally described as
Nocardia spp., Mycobacterium spp., and Corynebacterium spp.. Rhodococci are aero-
Table 7.1 Predominant bacteria in soil samples polluted with aliphatic and aromatic hydrocarbons, polycyclic aromatic hydrocarbons and chlorinated compounds.a
Gram-negative bacteria |
Gram-positive bacteria |
|
|
Pseudomonas spp. |
Nocardia spp. |
Acinetobacter spp. |
Mycobacterium spp. |
Alcaligenes sp. |
Corynebacterium spp. |
Flavobacterium/Cytophaga group |
Arthrobacter spp. |
Xanthomonas spp. |
Bacillus spp. |
aBacteria have been reclassified based on phylogenetic markers, resulting in changes in some genera and species; thus, the names of species are not mentioned.
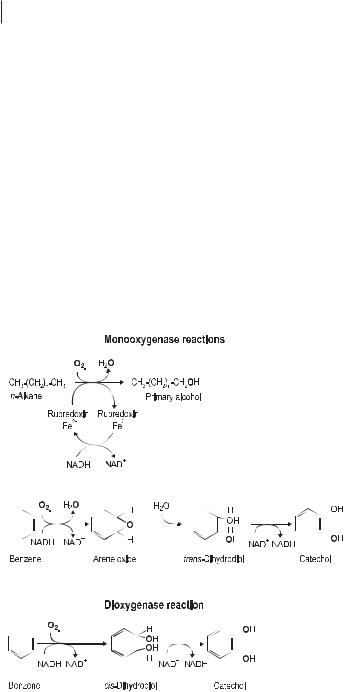
206 7 Aerobic Degradation of Recalcitrant Organic Compounds by Microorganisms
bic actinomycetes that show considerable morphological diversity. A certain group of these bacteria possesses mycolic acids on the external surface of the cell. These compounds are unusual long-chain alcohols and fatty acids, esterified to the peptidoglycan of the cell wall. These lipophilic cell structures probably are important for the affinity of rhodococci to lipophilic pollutants. In general, rhodococci have high and diverse metabolic activities and can synthesize biosurfactants.
7.2.2
Growth-associated Degradation of Aliphatics
The aerobic initial attack on aliphatic and cycloaliphatic hydrocarbons requires molecular oxygen. Two types of enzymatic reactions are involved in these processes (Fig. 7.2); whether a monooxygenase or a dioxygenase reaction occurs depends on the nature of the substrate and the enzymes possessed by the microorganisms. The n-alkanes are the main constituents of mineral oil contaminations. Long-chain n-al- kanes (C10–C24) are degraded most rapidly by the pathways shown in Figure 7.3. Short-chain alkanes (less than C9) are toxic to many microorganisms, but they evap-
Initial attack on xenobiotics by oxygenases. Monooxygenases incorporate one atom of dioxygen (O2) into the substrate, the second oxygen atom is reduced to H2O by means of reduction equivalents. Dioxygenases incorporate both atoms of O2 into the substrate.
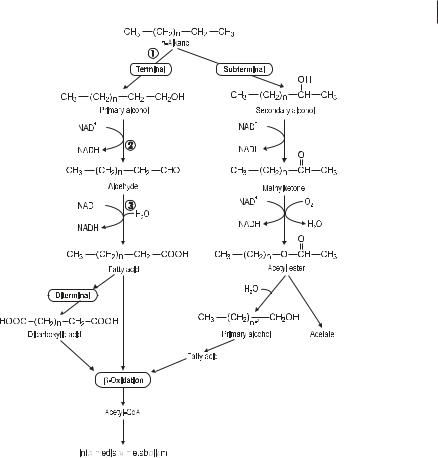
7.2 Principles of Bacterial Degradation 207
Pathways of alkane degradation. The main pathway is their terminal oxidation to fatty acids catalyzed by n-alkane monooxygenase, alcohol dehydrogenase, and ) aldehyde dehydrogenase.
orate rapidly from petroleum-contaminated sites. Oxidation of alkanes is classified as terminal or diterminal. Monoterminal oxidation is the main pathway and proceeds by formation of the corresponding alcohol, aldehyde, and fatty acid. Beta oxidation of fatty acids results in the formation of acetyl-CoA. n-Alkanes having an odd number of carbon atoms are degraded to propionyl-CoA, which is in turn carboxylated to methylmalonyl-CoA and further converted to succinyl-CoA. Fatty acids having a physiological chain length may be directly incorporated into membrane lipids, but most degradation products are fed into the tricarboxylic acid cycle. Subterminal oxidation occurs with lower (C3–C6) and longer alkanes, with formation of a secondary alcohol and subsequently of a ketone. Unsaturated 1-alkenes are oxidized at the saturated end of the chains. A minor pathway proceeds via an epoxide, which is converted to a fatty acid. Branching generally reduces the rate of biodegradation. Methyl side groups do not noticeably decrease the biodegradability, whereas complex
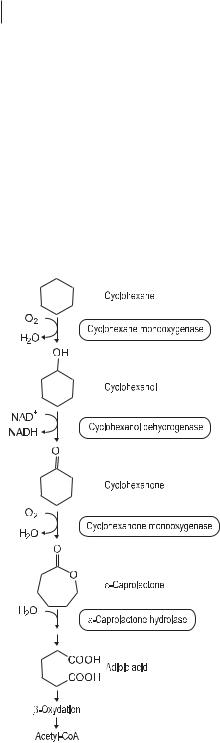
208 7 Aerobic Degradation of Recalcitrant Organic Compounds by Microorganisms
branched chains, e.g., the tertiary butyl group, hinder the action of the degradative enzymes.
Cyclic alkanes represent minor components of mineral oil and are relatively resistant to microbial attack. The absence of an exposed terminal methyl group complicates the primary attack. A few species can use cyclohexane as a sole carbon source, but it is more commonly cometabolized by mixed cultures. The pathway of cyclohexane degradation is shown in Figure 7.4. In general, the presence of alkyl sidechains on cycloalkanes facilitates their degradation.
Aliphatic hydrocarbons become less water soluble with increasing chain length; those with a chain length of C12 or more are virtually water-insoluble. Two mechanisms are involved in microbial uptake of these lipophilic substrates: attachment of microbial cells to oil droplets and production of biosurfactants. The uptake mechanism linked to attachment of the cells is still unknown, but the effect of biosurfac-
Fig. 7.4 Metabolic pathways for degradation of cycloaliphatic compounds (cycloparaffins).
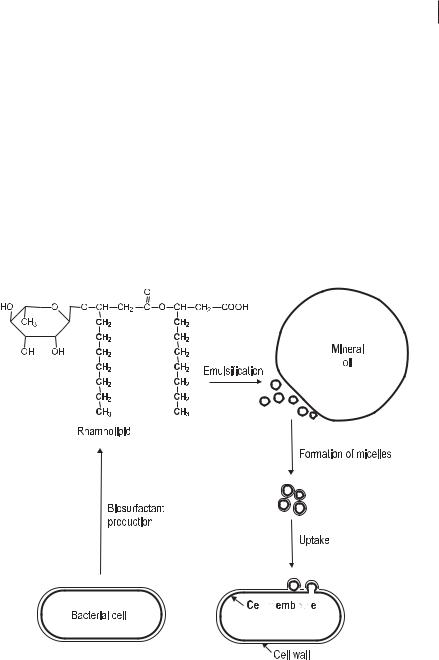
7.2 Principles of Bacterial Degradation 209
tants has been studied well (Fig. 7.5). Biosurfactants are molecules consisting of a hydrophilic and a lipophilic moiety. They act as emulsifying agents by decreasing the surface tension and by forming micelles. The latter can be encapsulated by the hydrophobic microbial cell surface.
The products of hydrocarbon degradation that are fed into the central tricarboxylic acid cycle have a dual function: as substrates of energy metabolism and building blocks for the biosynthesis of cell biomass (Fig. 7.1). Synthesis of amino acids and proteins requires nitrogen and sulfur sources, that of nucleotides and nucleic acids a phosphorous source. Biosynthesis of the bacterial cell wall requires activated sugars synthesized by gluconeogenesis. The cells act as complex biocatalysts of degradation.
Products of growth-associated degradation are CO2, H2O, and cell biomass. The cell biomass can be mineralized after exhaustion of degradable pollutants in a contaminated site.
Involvement of biosurfactants in the uptake of hydrocarbons. The emulsifying effect of a rhamnolipid produced by Pseudomonas spp. within the oil–water interphase and the formation of micelles are shown. Lipid phases are printed in bold.
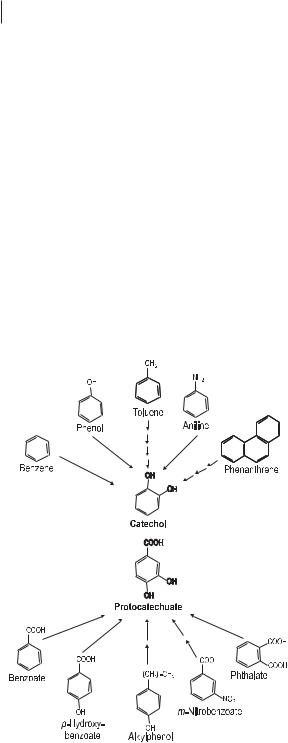
2107 Aerobic Degradation of Recalcitrant Organic Compounds by Microorganisms
7.2.3
Diversity of Aromatic Compounds: Unity of Catabolic Processes
Aromatic hydrocarbons, e.g., benzene, toluene, ethylbenzene and xylenes (BTEX compounds), and naphthalene, belong to the large-volume petrochemicals, which are widely used as fuels and industrial solvents. Phenols and chlorophenols are released into the environment as products and waste materials from industry. Aromatic compounds are formed in large amounts by all organisms, e.g., as aromatic amino acids, phenols, or hydroquinones/quinones. Thus, it is not surprising that many microorganisms have evolved catabolic pathways to degrade aromatic compounds. In general, man-made organic chemicals (xenobiotics) can be degraded by microorganisms when they are similar to natural compounds. The range of man-made aromatics shown in Figure 7.6 can be converted enzymatically to the natural intermediates of degradation: catechol and protecatechuate. In general, benzene and related compounds are characterized by greater thermodynamic stability than aliphatics are. The first step in benzene oxidation is a hydroxylation that is catalyzed by a dioxygenase (Fig. 7.2). The product, a diol, is then converted to catechol by a dehydrogenase. These initial reactions, hydroxylation and dehydrogenation, are also common
Degradation of a broad spectrum of natural and xenobiotic aromatic compounds into two central intermediates: catechol and protocatechuate.