
Environmental Biotechnology - Jordening and Winter
.pdf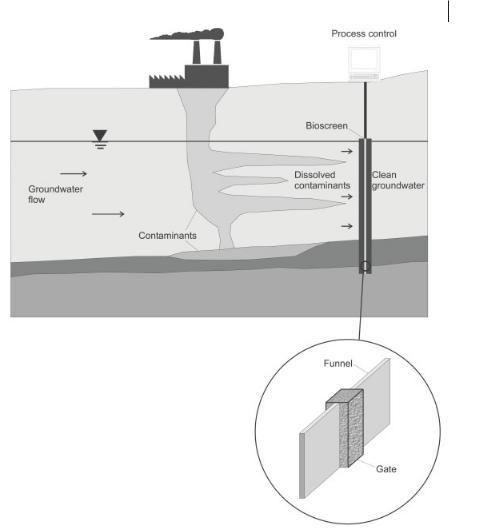
12.3 Remediation Technologies 325
Fig. 12.8 Process scheme of funnel-and-gate technology.
water flow velocity), as well as the biological degradation rates within individual redox zones, have to be determined by site-specific and/or laboratory investigations. These parameters and also the determined distribution of the contaminants are fed into the model. With the model the future extension of the plume can be estimated, allowing one to decide whether natural attenuation will be sufficiently effective. Experience has shown that natural attenuation is applicable in only a limited number of situations. However, it might be suitable as a post-treatment phase after active remediation. Natural attenuation already has been used to remove chlorinated ethenes, monoaromatic hydrocarbons (BTEX), and petroleum hydrocarbons [11].

326 12 In-situ Remediation
12.3.3.6 Evolving Technologies
Most of the evolving technologies have been investigated only on the laboratory scale, but some have already been tested on a pilot or even technical scale. However, detailed experience and wide commercial use are not available. Until now heavy metals have been treated with physicochemical technologies. Although these elements are not ‘degradable’, they are not biochemically inert. Several microbiological transformations are known that mainly alter the physicochemical behavior of metals [12], including
•solubilization and sorption (bioleaching, biosorption, phytoremediation)
•precipitation (bioprecipitation)
•volatilization by alkylation
Solubilization was originally developed to support the mining of metals (Me) by leaching (bioleaching) [13]. Various metabolites play an important role in bioleaching, e.g., surfactants, chelators, and organic acids. Many bacteria, e.g., Thiobacillus sp. and Leptospirillum ferrooxidans are reported to be able to leach metals. The metals, which occur mainly in solid ore deposits (MS2), are solubilized by two indirect mechanisms: via thiosulfate or via polysulfides and sulfur. Some metal sulfides are chemically attacked by Fe(III) hexahydrate ions, resulting in the formation of thiosulfate, which is oxidized to sulfuric acid. Other metal sulfides are attacked by Fe(III) and protons, which leads to the formation of polysulfides as intermediates and finally to elemental sulfur, which is biooxidized to sulfuric acid. The two mechanisms can be simplified by the following equations [14]:
• thiosulfate mechanism (FeS2, MoS2, WS2): |
|
FeS2 + 6 Fe3+ + 3 H2O → S2O32– + 7 Fe2+ + 6 H+ |
(1) |
S2O32– + 8 Fe3+ + 5 H2O → 2 SO42– + 8 Fe2+ + 10 H+ |
(2) |
• polysulfide mechanism (e.g., ZnS, CuFeS2, PbS): |
|
MeS + Fe3+ + H+ → Me2+ + 0.5 H2Sn + Fe2+ (n ≥ 2) |
(3) |
0.5 H2Sn + Fe3+ → 0.125 S8 + Fe2+ + H+ |
(4) |
0.125S8 + 1.5 O2 + H2O → SO42– + 2 H+ |
(5) |
The bioleaching method requires pumping of the groundwater and removal of the solubilized metals in a groundwater treatment plant. For treatment, biosorption may be used. In summary, although bioleaching is a pump-and-treat process, the higher solubility of the ‘contaminants’, i.e., the solubilized metals, may result in enhanced removal of the contaminants compared to classical pump-and-treat procedures.
Another technology for removing dissolved contaminants from soil and groundwater is phytoremediation: contaminants are taken up through the roots of plants and trees. The choice of plants depends on the characteristics of the contaminants and the soil as well as on the three-dimensional distribution of the contaminants, be-

12.3 Remediation Technologies 327
cause the efficiency of phytoremediation is restricted to the depth of root growth. Some contaminants, e.g., nitroaromatics, are taken up but are not transported within the plant. Transformation of the contaminants is usually not effective enough for final elimination and is therefore of minor importance. For example, heavy metals can be accumulated without significant transformation by some plants to a very high extent. Hence, for final removal of the contaminants, the plants have to be harvested and eliminated. Because of the high water demand of some trees, phytoremediation can also be used for a hydraulic limitation of a pollution plume.
A main problem in the mining industry is acidification and contamination of groundwater with dissolved metal ions together with sulfate. With bioprecipitation, both problems can be solved together. Addition of an organic substrate (represented by ‘CH2O’) leads to the formation of sulfide and an increase in the pH. The metals (Me2+) are precipitated as a nontoxic metal sulfide in an abiotic reaction:
SO42– + CH2O → H2S + HCO3– |
(6) |
Me2+ + H2S → MeS↓ + H+ |
(7) |
Many metals can also be precipitated as metal carbonates or hydroxides. The reactions are disturbed by the presence of oxygen. However, O2 is readily consumed when an organic substrate is added. Most formed metal sulfides are very stable even if the redox milieu recovers after finalization of the organic substrate addition. Remobilization can occur when the pH drops to low values. If excess formation of H2S occurs, a second, aerobic treatment phase is required, in which surplus H2S is reoxidized to sulfate. With bioprecipitation, contamination with at least the following metals can be treated: Pb, Zn, Cu, Cd, Ni. The mobile, very toxic, hexavalent chromium ion (Cr6+) has been reported to serve as an alternative electron acceptor for dissimilatory Fe(III)-reducing bacteria, which use reduction of Cr6+ to the less mobile, nontoxic, trivalent chromium (Cr3+), which precipitates as chromium hydroxide to gain energy for growth. Chromium hydroxide is stable also in aerobic environments. Comparable reactions are reported for uranium (U6+). Fe(II) and S2– can also reduce Cr(VI) in nonenzymatic reactions.
In addition to these redox reactions, microorganisms can form and degrade organometals. Alkylation and dealkylation are carried out by a wide range of microorganisms, which changes several important parameters such as toxicity, volatility, and water solubility of the contaminants [15]. For example, arsenic can be removed from the vadose zone by this bioprocess. For microorganisms this process is linked to detoxification of the environment, because the volatile transformation products evaporate readily into the atmosphere. However, the volatile products are rather toxic. Therefore, using this bioprocess as a remediation technology requires extraction of the volatile products (e.g., by soil vapor extraction). The extracted contaminated soil vapor can be treated chemically: the compound is dealkylated and the product is removed in a gas scrubber. The following microbial pathways for reducing inorganic arsenic to volatile organic compounds can occur. Arsenate (As5+) and arsenite (As3+) are transformed to arsine (AsH3) by bacteria, preferably under anaerobic conditions.

328 12 In-situ Remediation
Fungi and some bacteria transform arsenate and arsenite to diand trimethylarsine in an aerobic environment. The process can take place only when an appropriate C source is available.
As5+, As3+ + Corg → (CH3)2As + (CH3)3As |
(8) |
In addition, several other biotransformation reactions forming a variety of other products can occur. For example, As5+ can be consumed as an electron acceptor during the oxidation of organic matter, resulting in its reduction to the more soluble and more toxic As3+ [2]. Until now, the necessary environmental conditions for a controlled microbial arsenic volatilization have not been investigated. Although such reduction processes (leading to gaseous As3– compounds) are well documented from laboratory experiments to occur with significant transformation rates, substantial natural arsenic losses in the field have not yet been observed [16]. The bioprocess that adds methyl groups to metal ions, forming volatile organometals that volatilize from the environmental compartment, is a general detoxification process used by bacteria. It is well documented, e.g., for mercury (Hg) [17].
Concerning radioactive metals, we should note that a bacterium, Deinococcus radiodurans, was isolated that transforms, e.g., toluene, even under high doses of ã radiation. Only the organic pollutants were degraded; the radionuclide of course remained unaffected. The survival of this microorganism in a radioactive environment led to speculation that probably some as-yet unknown bacteria exist which will allow complete removal of radionuclides from the environment by bioleaching. Future progress may also be achieved by constructing genetically engineered microorganisms (GEM) that contain, e.g., a complete degradation sequence for final mineralization of contaminants, specific genes to resist unfavorable environments, or other improved biochemical features, like a deficiency in adhesion, which will be useful for bioaugmentation.
12.4 Monitoring
The conditions for the success of a remediation measure are the controllability of its bioprocesses and the technological skills of the operators. The reactions occurring within an aquifer during in situ microbiological remediation are manifold, including both abiotic and biotic processes. In situ bioremediation can be successful only if the transport of nutrients and electron acceptors and donors to the contaminants and the removal of metabolic end products (e.g., CH4, N2) is sufficient. Otherwise, gas bubbles can form, resulting in a change in the transport process. For this reason, hydrogeological parameters have to be included in the monitoring. Remediation can be successful only if it is accompanied by close monitoring. The tasks of monitoring are therefore to obtain information for the following purposes:
•controlling the addition of electron acceptors, donors, and nutrient salts
•controlling biogeochemical site conditions

12.4 Monitoring 329
•demonstrating that remediation processes are occurring
•indicating when the remediation target has been reached
In a continuous remediation process, the monitoring data are used to optimize the bioprocess and to correct malfunctions.
When unsaturated soil is treated, the most important monitoring instrument is the in situ respiration detector, which determines the consumption of oxygen and formation of carbon dioxide in the soil vapor without soil vapor extraction. Because CO2 formation can be influenced by abiotic CO2 fixation within the soil matrix (e.g., as carbonates), only O2 consumption allows good determination of the actual degradation rate with the help of stoichiometric factors. That is, we know that the mineralization of 1 g of mineral oil hydrocarbons requires the consumption of 3.5 g of O2 (neglecting biomass formation). The change in the degradation rate with time allows assessment of the quality of the installed remediation measures. A decrease in the degradation rate may indicate a lack of water, nutrients, or degradable contaminants.
Monitoring remediation measures in saturated soil is often more complex. Usually, groundwater samples are taken and the following parameters are determined:
•contaminants
•metabolites (specific or as dissolved organic carbon, DOC)
•degradation end products (CO2, CH4, ethene, ethane)
•nutrient salts (e.g., NH4+, PO43– )
•electron acceptors (O2, NO3–, Fe(III), Mn(IV), SO42– )
•reduced electron acceptor species (NO2–, Fe(II), Mn(II), S2– )
•electron donors
•field parameters (pH, redox potential, electrical conductivity, temperature)
•optional bacterial counts (total counts, contaminant degraders, denitrifiers)
Because of the variety of possible metabolites, it is usually not possible to determine specific compounds separately; they are usually summarized as DOC. Sometimes (e.g., when water treatment plants are involved in the remediation process), more detailed information on the character of the DOC is required. This information can be supplied by LC–OCD (liquid chromatography with organic carbon detection) analysis, by which the DOC is divided into fractions of humic substances, building blocks, low molecular weight acids, amphiphilic substances, and polysaccharides. Monitoring the degradation end products is necessary if balancing of the remediation is required and complete biodegradation must be shown. However, until now no reliable methods for balancing technical-scale in situ processes are available. Usually a more pragmatically approach is chosen, i.e., only a limited number of parameters are determined. These parameters include monitoring the nutrients and electron acceptors or electron donors (in anaerobic processes) to ensure a sufficient supply of these supplements. The field parameters are monitored to ensure that the remediation measures have led to environmental conditions optimal for contaminant degradation. Here, an increase in the counts of specific contaminant-degrading bacteria is expected. However, investigations have shown that remediation meas-

330 12 In-situ Remediation
ures strongly interfere with the indigenous microflora, changing the biodiversity in complex ways. The effects are not yet well understood.
Because many in situ technologies are based on controlled hydraulic conditions, it is evident that maintenance of the designed infiltration rate or groundwater flow rate is of utmost importance. Most pollutants exhibit low solubility and a high tendency to bind to the soil matrix, resulting in strong retardation of their transport. Elimination of the contaminants is enhanced by biodegradation at the place where the contaminants are located. Hence, transport of nutrients and electron acceptors and donors to the contaminants is necessary. Once the pollutants are solubilized, they are degraded prior to resorption. Thus, biodegradation increases the concentration gradient between sorbed/solid and dissolved substances, which accelerates desorption, dissolution, and diffusion of pollutants out of micropores (where they are not available for biodegradation). Changing the hydraulic conductivity of the aquifer strongly affects the progress of remediation. Hence, monitoring the hydraulic conductivity is necessary. A decrease in hydraulic conductivity can be caused by formation of gas bubbles (due to excess infiltration of H2O2 or high denitrification rates), precipitation of Fe oxide (during changing redox conditions), clogging with produced biomass, or shifting of fine soil particles caused by fast infiltration. By correlating an observed decrease in hydraulic conductivity with actual remediation measures, the influence of the remediation on the hydraulic conductivity can be minimized.
Generally, owing to the partitioning equilibrium of soluble gases between groundwater and soil vapor, monitoring of volatile contaminants, degradation end products (CO2, CH4), electron acceptor (O2), and natural tracer radon (222Rn) can be used to control the remediation. Degradation end products are monitored to balance the in situ processes. Monitoring O2 can prevent oversaturation with respect to this electron acceptor. By monitoring the natural tracer radon, transport from groundwater to soil vapor and from soil vapor to the atmosphere and also dilution of soil vapor with atmospheric air can be determined.
12.5 Outlook
Until now various in situ remediation technologies have been developed, and the growing experience with in situ technologies as well as ongoing research today allows many processes that occur in the subsurface to be predicted during remediation planning. This results in the application of economic remediation processes. Recent developments have considered natural limitations in a much better way than before, resulting in increased use of slower processes like the passive technologies. There is still a large potential for development of in situ technologies. Although today more efforts are made in environmental protection management, the current environmental situation shows that end-of-the-pipe technologies will still be needed for decades.

References 331
References
1Klein, J., Dott, W., Laboratory Methods for the Evaluation of Biological Soil Cleanup Processes. Frankfurt 1992: Dechema.
2Anderson, R. T., Lovley, D. R., Ecology and biogeochemistry of in situ groundwater bioremediation, in: Advances in Microbial Ecology Vol. 15 (Jones, ed.), pp. 289–350. New York 1997: Plenum.
3 Nyer E. K., Palmer, P. L., Carman, E. P., Boettcher, G., Bedessem, J. M., Lenzo, F., Crossman, T. L., Rorech, G. J., Kidd, D., In Situ Treatment Technology. Boca Raton, FL 2001: CRC Lewis.
4 Rogers, S. W., Ong, S. K., Influence of porous media, airflow rate and air channel spacing on benzene NAPL removal during air sparging. Environ. Sci. Technol. 2000, 34, 764–770.
5 Reddy, K. R., Kosgi, S., Zhou, J., A review of in situ air sparging for the remediation of VOC-contaminated saturated soils and groundwater, Hazardous Waste
– Hazardous Materials 1995, 12, 97–117.
6 Rosansky, S. H., Kramer, J., Coonfare, C., Wickramanayake, G. B., Moring, B. K., Maughon, M. J., Casley, M. J., Optimization and monitoring of bioslurping systems for free-product recovery, in:
Risk, Regulatory, and Monitoring Considerations: Remediation of Chlorinated and Recalcitrant Compounds (Wickramanayake, G. B., Gavaskar, A. R., Kelley, M. E., Nehring, K. W., eds.), pp. 339–348. Columbus, OH 2000: Battelle Press.
7 Spuij, F., Alphenaar, A., de Wit, H., Lubbers, R., v/d Brink, K. et al., Full-scale application of in situ bioremediation of PCE-contaminated soil, in: Pap. 4th Int. In Situ On-Site Biorem. Symp. 4, pp. 431–437. Columbus, OH 1997: Battelle Press.
8 Rijnaarts, H. H. M., Brunia, A., Van Aalst, M., In situ bioscreens, in: Pap. 4th Int. In Situ On-Site Biorem. Symp. 4, pp. 203–208. Columbus, OH 1997: Battelle Press.
9Robertson, W. D., Cherry, J. A., Long-term performance of the Waterloo denitrification barrier, Land Contam. Reclam. 1997, 5, 183–188.
10Benner, S. G., Blowes, D. W., Ptacek, C. J., A full-scale porous reactive wall for prevention of acid mine drainage, Ground Water Monit. Rem. 1997, 17, 99–107.
11Wiedemeier, T. H., Wilson, J. T., Kampbell, D. H., Miller, R. N., Hansen, J. E., Technical protocol for implementing intrinsic remediation with long-term monitoring for natural attenuation of fuel contamination dissolved in groundwater,
AFC Environ. Excellence Books. 1995: AFB, San Antonio, Texas.
12Singh, B., Wilson, M. J., Geochemistry of acid mine waters and the role of microorganisms in such environments: a review,
Adv. Geoecol. 1997, 30, 159–192.
13Rawlings, D. E., Silver, S., Mining with microbes. Biotechnology 1995, 13, 773–778.
14Schippers, A., Sand, W. Bacterial leaching of metal sulfides proceeds by two indirect mechanisms: via thiosulfate or via polysulfides and sulfur, Appl. Environ. Microbiol.
1999, 65, 319–321.
15White, C., Gadd, G. M., Reduction of metal cations and oxyanions by anaerobic and metal-resistant microorganisms: chemistry, physiology, and potential for the control and bioremediation of toxic metal pollution, in: Extremophiles: Microbial Life in Extreme Environments (Horikoshi, K., Grant, W. D., eds.), pp. 233–254. New York 1998: Wiley-Liss.
16Stoeppler, M., Arsenic, in: Elements and Their Compounds in the Environment. Occurrence, Analysis and Biological Relevance, 2nd Ed. (Merian, E., Anke, M., Ihnat, M., Stoeppler, M. eds.), pp. 1321–1364. Weinheim 2004: Wiley-VCH.
17Matilainen, T., Involvement of bacteria in methyl mercury formation in anaerobic lake waters, Water Air Soil Pollut. 1995, 80, 757–764.

333
13
Composting of Organic Waste
Frank Schuchardt
13.1 Introduction
Composting is the biological decomposition of the organic compounds of wastes under controlled aerobic conditions. In contrast to uncontrolled natural decomposition of organic compounds, the temperature in waste heaps can increase by selfheating to the ranges of mesophilic (25–40 °C) and thermophilic microorganisms (50–70 °C). The end product of composting is a biologically stable humus-like product for use as a soil conditioner, fertilizer, biofilter material, or fuel.
The objectives of composting can be stabilization, volume and mass reduction, drying, elimination of phytotoxic substances and undesired seeds and plant parts, and sanitation. Composting is also a method for decontamination of polluted soils. Almost any organic waste can be treated by this method. The pretreatment of organic waste by composting before landfilling can reduce the emissions of greenhouse gases.
In any event, composting of wastes is conducted with the objective of high economic effectiveness and has the goal of compost production with the lowest input of work and expenditure. The consequence of this approach is the effort to optimize the biological, technical, and organizational factors and elements that influence the composting process. The factors that influence the composting process are well known and have been published in numerous reviews and monographs. The period since 1970 has been characterized by the development of new strategies, composting processes, and technologies and the optimization of existing processes against the background of an expanding market for composting technology. Among others, reasons for these developments are rising costs for sanitary landfills, improved environmental protection requirements, as well as new laws, ordinances, and regulations. The realization that resources are limited and the idea of recycling refuse back to soil have also provided important impetuses for developments in this field.
Numerous publications and reviews on composting are published in specific journals such as Compost Science and Utilization, Bioresource Technology, and Biosystems Engineering. Some monographs give an overview of the field of composting [1–8].
Environmental Biotechnology. Concepts and Applications. Edited by H.-J. Jördening and J. Winter Copyright © 2005 WILEY-VCH Verlag GmbH & Co. KGaA, Weinheim
ISBN: 3-527-30585-8
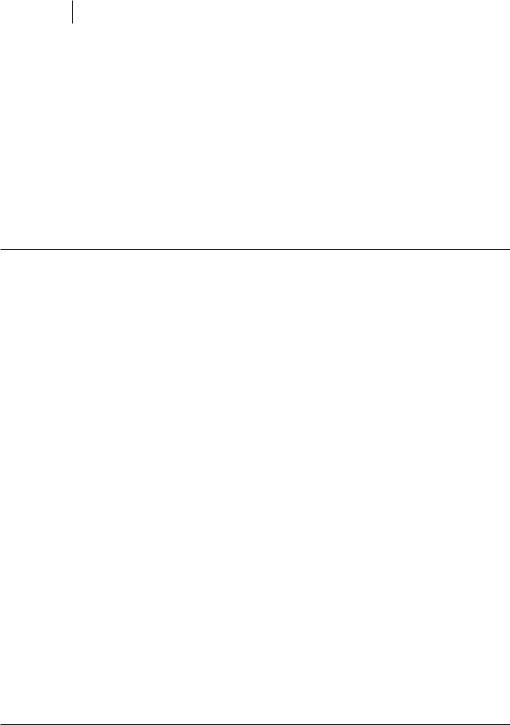
33413 Composting of Organic Waste
13.2
Waste Materials for Composting
The origins of organic waste for composting are households, industry, wastewater treatment plants, agriculture, horticulture, landscapes, and forestry (Table 13.1). The amount, composition, and physical characteristics of plant wastes are influenced by numerous factors such as the origin, production process, preparation, season, collecting system, social structure, and culture. The wide range in the amount and composition of waste requires analyses for planning a composting plant and for estimating the compost quality in each individual situation.
Table 13.1 Nutrient content per dry weight of some wastes for composting [3; own analyses].
Waste |
VSa |
C/N |
N |
P2O5 |
K2O |
CaO |
MgO |
|
[%] |
[–] |
[%] |
[%] |
[%] |
[%] |
[%] |
|
|
|
|
|
|
|
|
Kitchen waste |
20–80 |
12–20 |
0.6–2.2 |
0.3–1.5 |
0.4–1.8 |
0.5–4.8 |
0.5–2.1 |
Biowaste |
30–70 |
10–25 |
0.6–2.7 |
0.4–1.4 |
0.5–1.6 |
0.5–5.5 |
0.5–2.0 |
Garden and green waste |
15–75 |
20–60 |
0.3–2.0 |
0.1–2.3 |
0.4–3.4 |
0.4–12 |
0.2–1.5 |
Garbage |
25–50 |
30–40 |
0.8-1.1 |
0.6–0.8 |
0.5–0.6 |
4.4-5.6 |
0.8 |
Feces (human) |
15–25 |
6–10 |
2 |
1.8 |
0.4 |
5.4 |
2.1 |
Wastewater sludge (raw) |
20–70 |
15 |
4.5 |
2.3 |
0.5 |
2.7 |
0.6 |
Wastewater sludge |
|
|
|
|
|
|
|
(anaerobic stabilized) |
15–30 |
15 |
2.3 |
1.5 |
0.5 |
5.7 |
1.0 |
Dung |
|
|
|
|
|
|
|
Cattle |
20.3 |
20 |
0.6 |
0.4 |
0.7 |
0.6 |
0.2 |
Horses |
25.4 |
25 |
0.7 |
0.3 |
0.8 |
0.4 |
0.2 |
Sheep |
31.8 |
15–18 |
0.9 |
0.3 |
0.8 |
0.4 |
0.2 |
Pigs |
18.0 |
15–20 |
0.8 |
0.9 |
0.5 |
0.8 |
0.2 |
Liquid manure |
|
|
|
|
|
|
|
Cattle |
10–16 |
8–13 |
3.2 |
1.7 |
3.9 |
1.8 |
0.6 |
Pigs |
10–20 |
5–7 |
5.7 |
3.9 |
3.3 |
3.7 |
1.2 |
Chickens |
10–15 |
5–7 |
9.8 |
8.3 |
4.8 |
17.3 |
1.7 |
Beet leaves |
70 |
15 |
2.3 |
0.6 |
4.2 |
1.6 |
1.2 |
Straw |
90 |
100 |
0.4 |
2.3 |
2.1 |
0.4 |
0.2 |
Bark, fresh |
90–93 |
85–180 |
0.5–1.0 |
0.02–0.06 |
0.03–0.06 |
0.5–1 |
0.04–0.1 |
Bark mulch |
60–85 |
100–130 |
0.2–0.6 |
0.1–0.2 |
0.3–1.5 |
0.4–1.3 |
0.1–0.2 |
Wood chips |
65–85 |
400–500 |
0.1–0.4 |
1.0 |
0.3–0.5 |
0.5–1.0 |
0.1–0.15 |
Leaves |
80 |
20–60 |
0.2–0.5 |
— |
— |
— |
— |
Reed |
75 |
20–50 |
0.4 |
— |
— |
— |
— |
Peat |
95–99 |
30–100 |
0.6 |
0.1 |
0.03 |
0.25 |
0.1 |
Paunch manure |
8.5–17 |
15–18 |
1.4 |
0.6 |
0.9 |
2.0 |
0.6 |
Grape marc |
81 |
50 |
1.5–2.5 |
1.0–1.7 |
3.4–5.3 |
1.4–2.4 |
0.2 |
Fruit marc |
90–95 |
35 |
1.1 |
0.6 |
1.6 |
1.1 |
0.2 |
Tobacco |
85–88 |
50 |
2.0–2.4 |
0.5–6.6 |
5.1–6.0 |
5.0 |
0.1–0.4 |
Paper |
75 |
170–180 |
0.2–1.5 |
0.2–0.6 |
0.02–0.1 |
0.5–1.5 |
0.1–0.4 |
a VS Volatile solids.

13.3 Fundamentals of Composting Process 335
Table 13.2 Heavy metal content per dry weight of some wastes for composting [4].
Waste |
Zn |
Cu |
Cd |
Cr |
Pb |
Ni |
Hg |
|
[mg kg–1] |
[mg kg–1] |
[mg kg–1] |
[mg kg–1] |
[mg kg–1] |
[mg kg–1] |
[mg kg–1] |
|
|
|
|
|
|
|
|
Biowaste |
50–470 |
8–81 |
0.1–1 |
5–130 |
10–183 |
6–59 |
0.01–0.8 |
Green waste |
30–138 |
5–31 |
0.2–0.9 |
28–86 |
24–138 |
9–27 |
0.1–3.5 |
Paper |
93 |
60 |
0.2 |
4 |
20 |
1 |
0.08 |
Paper, printed |
112 |
66 |
0.2 |
31 |
78 |
3 |
0.04 |
Paper, collected |
40 |
21 |
0.2 |
3 |
12 |
1 |
0.06 |
Paper sludge |
150–1500 |
15–100 |
0.1–1.5 |
30–300 |
70–90 |
5–15 |
0.2–0.5 |
Bark |
150–300 |
40–60 |
0.6–2.1 |
30–63 |
20–57 |
12–20 |
0.1–0.5 |
Bark mulch |
40–500 |
10–30 |
0.1–2 |
500–1000 |
50–100 |
30–60 |
0.1–1 |
Wood chips |
58–137 |
8–11 |
0.1–0.2 |
6–8 |
13–53 |
4 |
0.1 |
Grapes marc |
60–80 |
100–200 |
0.5 |
2.5–7.4 |
10 |
1–4 |
0.02–0.04 |
Fruit marc |
20–30 |
9.5 |
0.2 |
0.02–1 |
0.3–1 |
2–4 |
0.03 |
Brewers’ grains |
13 |
6 |
0.3 |
16 |
10 |
16 |
0.04 |
Oil seed residues |
4 |
1 |
0.03–0.05 |
0.1 |
0.1–0.4 |
1–3 |
0.01 |
Cacao hulls |
89 |
7–12 |
0.25 |
0.5 |
0.4 |
0.3 |
0.02 |
|
|
|
|
|
|
|
|
The content of heavy metals and organic compounds in the waste is of great importance, particularly with regard to the use of compost as soil conditioner and fertilizer (Table 13.2). Ways to reduce the heavy metal content in compost from biowaste are to collect the waste separately and to obtain detailed information of the producers of the waste. The fact that the concentrations of heavy metals increase as the organic compounds are degraded also must be taken into consideration.
Analyses of biowaste show a content of impurities (e.g., plastics, glass, metals, rocks) between 0.5% and 5.0%, depending on the social structure, buildings, and public relations work in the area [2]. In densely built-up areas the contamination is higher than in others. More than 90% of the impurities have a size >60 mm, and 90% of the biowaste materials have a size <60 mm [1].
Depending on climatic and cultural conditions (e.g., growing and harvest times, holidays, traditions) some plant wastes, like leaves or branches, are not available during the whole year. An important factor for the operation of a composting plant can be the fluctuation in the composition of the wastes, in particular, the water content [5].
13.3
Fundamentals of Composting Process
Degradation of the organic compounds in waste during composting is initiated predominately by a very diverse community of microorganisms: bacteria, actinomyctes, and fungi [7, 9, 10, 18, 23, 24, 28, 33, 36]. Just as in biological wastewater treatment, an additional inoculum for the composting process is not generally necessary, because of the high number of microorganisms in the waste itself and their short gen-