
- •Contents
- •Preface
- •1 Introduction: the significance of radiobiology and radiotherapy for cancer treatment
- •2 Irradiation-induced damage and the DNA damage response
- •3 Cell death after irradiation: how, when and why cells die
- •4 Quantifying cell kill and cell survival
- •5 Dose–response relationships in radiotherapy
- •6 Linear energy transfer and relative biological effectiveness
- •7 Tumour growth and response to radiation
- •8 Fractionation: the linear-quadratic approach
- •9 The linear-quadratic approach in clinical practice
- •10 Modified fractionation
- •11 Time factors in normal-tissue responses to irradiation
- •12 The dose-rate effect
- •13 Pathogenesis of normal-tissue side-effects
- •14 The volume effect in radiotherapy
- •15 The oxygen effect and fractionated radiotherapy
- •16 The tumour microenvironment and cellular hypoxia responses
- •17 Therapeutic approaches to tumour hypoxia
- •18 Combined radiotherapy and chemotherapy
- •19 Retreatment tolerance of normal tissues
- •20 Molecular image-guided radiotherapy with positron emission tomography
- •21 Molecular-targeted agents for enhancing tumour response
- •22 Biological response modifiers: normal tissues
- •23 Molecular targeting and patient individualization
- •24 Protons and other ions in radiotherapy
- •25 Second cancers after radiotherapy
- •Glossary of terms in radiation biology
- •Index

24
Protons and other ions in radiotherapy
WOLFGANG DÖRR AND MICHAEL C. JOINER
24.1 |
Introduction |
332 |
24.6 |
Second cancers |
336 |
24.2 |
Biological characteristics of high-linear |
|
24.7 |
Ion radiotherapy in paediatric oncology |
336 |
|
energy transfer (LET) beams |
332 |
24.8 |
Conclusions and future directions |
336 |
24.3 |
Dose specification and ion |
|
Key points |
337 |
|
|
radiotherapy planning |
334 |
Bibliography |
337 |
|
24.4 |
Radiotherapy with protons |
335 |
Further reading |
338 |
|
24.5 |
Radiotherapy with heavy ions |
336 |
|
|
|
|
|
|
|
|
|
24.1 INTRODUCTION
The physical characteristics of light and heavy ion radiation beams, as well as of neutrons, were introduced in Chapter 6. In this chapter, we will review the biological properties of ions (and neutrons) with relevance to radiotherapy, and summarize the recent experience and status of proton and heavy ion radiotherapy.
The major difference between radiotherapy with photons and ions is in the spatial distribution of physical dose. For photons, independent of energy, the maximum dose is deposited close to the entrance surface of the matter that is penetrated. In contrast, ions enter tissue with a low dose and the maximum dose deposition occurs within the socalled Bragg peak, at a depth depending on the beam energy. Behind this Bragg peak region, for protons no significant further dose is deposited, and in the case of heavy ions only a minor dose, owing to some nuclear fragments, is deposited.
A second advantage of ions over megavoltage X-rays is the steep dose gradient at the beam margins, with a reduction from 90 per cent to 10 per cent within few millimetres. This sharpness in the beam definition is even more pronounced for heavy ions than for protons, by a factor of approximately three. Moreover, in the case of heavy ions,
a small amount of positron emission (11C, 15O) is generated via nuclear reactions, and this can be exploited to visualize the dose distribution by positron emission tomography (PET).
24.2 BIOLOGICAL CHARACTERISTICS OF HIGH-LINEAR ENERGY TRANSFER (LET) BEAMS
Figure 24.1 illustrates that only minor changes occur in the total dose required for various biological effects (acutely responding tissues indicated with dashed lines and late-responding tissues with solid lines) with changes in dose per fraction for high-LET radiation (neutrons). Compared with low-LET radiation, particularly for late tissue endpoints, these changes are significantly less pronounced (Withers et al., 1982). Figure 24.1 should be compared with Fig. 8.1, which shows these relationships for a similar range of tissues exposed to fractionated low-LET radiation. This comparison is illustrated schematically in Fig. 24.2 and the following conclusions can be drawn:
1There is much less effect of dose fractionation (i.e. of dose per fraction) for high-LET radiation, either in acutely responding or in
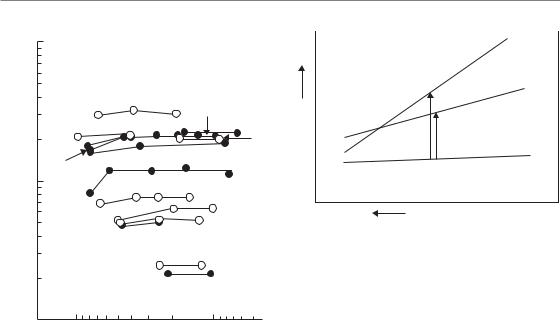
Biological characteristics of high-linear energy transfer 333
|
100 |
|
Neutrons |
|
|
|
|
|
|
||
|
50 |
|
|
|
|
(Gy) |
|
|
15 |
10 |
|
isoeffects |
20 |
12 |
13 |
14 |
9 |
|
|
||||
|
|
|
|||
11 |
|
8 |
|
|
|
|
|
7 |
|
||
for various |
|
|
|
||
|
|
|
|
||
10 |
|
|
6 |
|
|
|
|
|
|
||
|
|
|
5 |
|
|
dose |
5 |
|
3 |
4 |
|
|
|
|
|
||
|
|
|
|
|
|
Total |
|
|
|
2 |
|
|
2 |
|
|
1 |
|
|
1 |
5 |
2 |
1 |
0.5 |
|
10 |
||||
|
|
Dose per fraction (Gy) |
|
Figure 24.1 Summary of published data on isoeffect curves for neutrons as a function of dose per fraction in various tissues of mice and rats. Broken lines indicate data on acute-responding tissues; solid lines are for late-responding tissues. Compare with Fig. 8.1. Key: 1, thyroid function; 2, haemopoietic colonies; 3, vertebral growth; 4, spermatogenic colonies; 5, fibrosarcomas; 6, jejunum colonies; 7, lung LD50; 8, lumbar nerve root function; 9 and 12, skin desquamation; 10, skin contraction; 11, skin late changes; 13, spinal cord; 14, oral mucosa necrosis; 15, skin necrosis. From Withers et al. (1982), with permission.
late-responding tissues (Fig. 24.1). Tissue responses to high-LET radiations (heavy ions, neutrons) therefore demonstrate substantially higher α/β ratios than low-LET (photon) beam responses, as is also the case for cell lines in vitro (see Fig. 6.2).
2.For photons, the total dose increases more steeply with decreasing dose per fraction for
late-responding than for early-responding tissues, reflecting the smaller α/β ratios for lateresponding tissues (see Chapters 8 and 9). The relative biological effectiveness (RBE) therefore rises rapidly with decreasing dose per fraction for late-responding tissues and more gradually for early-responding tissues.
|
|
Low-LET, |
|
|
|
late-responding |
|
|
|
tissues |
|
(Gy) |
RBElate |
Low-LET, |
|
|
|||
RBEearly |
early-responding |
||
tissues, tumours |
|||
Total dose |
|||
|
High-LET, all tissues |
||
|
|
Dose per fraction (Gy)
Figure 24.2 Illustration of the changes in isoeffective total doses with decreasing dose per fraction for acuteand late-responding tissues and tumours, for irradiation with high-linear energy transfer (LET) or low-LET radiation, respectively. The corresponding definition of relative biological effectiveness (RBE) values is indicated.
3.The RBE values for late tissue responses are not intrinsically higher than for acute responses. However, because of their faster increase as dose is reduced, the RBE values for late tissue responses tend to be higher than for early tissue response at lower doses per fraction, especially at or below 2 Gy per (X-ray) fraction.
To further illustrate this last point, Fig. 24.3 demonstrates the rise in neutron RBE (compared with photons) with decreasing dose per fraction in epidermis, as an example of an early-responding tissue, and kidney (a late-responding tissue). In this example, the RBE for d(16)-Be neutrons in kidney was greater than in epidermis at an X-ray dose per fraction of 2 Gy, but lower for a more highly penetrating p(62)-Be neutron therapy beam. Therefore, compared with conventional photon therapy, late renal damage would be increased more substantially relative to early reactions (and perhaps relative to tumour response) by treating with a low-energy neutron beam, but late renal injury would actually be spared with the high-energy neutron radiation.
It must be emphasized that these relationships are clearly specific for the tissues and the high-LET beams studied. Similar relationships between other earlyand late-responding tissues may not follow
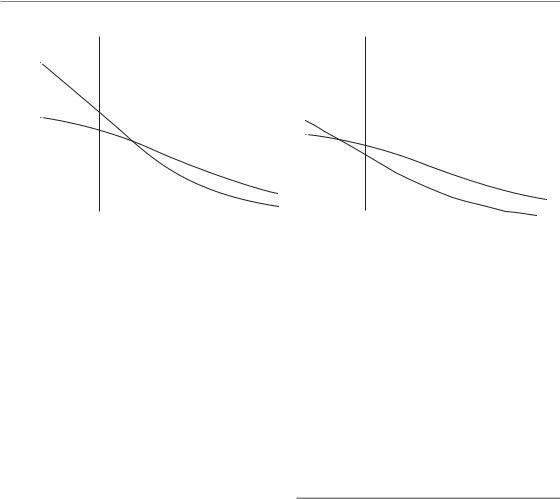
334 Protons and other ions in radiotherapy
Relative biological effectiveness
(a)
7 |
|
|
|
|
|
|
|
|
|
|
|
|
|
|
|
|
|
|
|
7 |
|
|
|
|
|
|
|
|
|
|
|
|
|
|
|
|
|
|
|
|
|
|
|
|
|
|
|
|
|
|
|
|
|
|
|
|
|
|
|
|
|
|
|
|
|
|
|
|
|
|
|
|
|
|
|
|
|
|
|
|
|
|
|
||
6 |
|
|
|
|
|
|
|
|
|
|
|
|
|
|
|
|
|
|
|
6 |
|
|
|
|
|
|
|
|
|
|
|
|
|
|
|
|
|
|
|
|
|
|
|
|
|
|
|
|
|
|
|
|
|
|
|
|
|
|
|
|
|
|
|
|
|
|
|
|
|
|
|
|
|
|
|
|
|
|
|
|
|
|
|
||
|
|
|
|
|
|
|
|
|
|
|
|
|
|
|
|
|
|
|
|
|
|
|
|
|
|
|
|
|
|
|
|
|
|
|
|
|
|
|
|
|
||
5 |
|
|
|
|
|
|
|
|
|
|
|
|
|
|
|
|
|
|
|
5 |
|
|
|
|
|
|
|
|
|
|
|
|
|
|
|
|
|
|
|
|
|
|
|
|
|
|
|
|
|
|
|
|
|
|
|
|
|
|
|
|
|
|
|
|
|
|
|
|
|
|
|
|
|
|
|
|
|
|
|
|
|
|
|
||
|
|
|
|
|
|
|
|
|
|
|
|
|
|
|
|
|
|
|
|
|
|
|
|
|
|
|
|
|
|
|
|
|
|
|
|
|
|
|
|
|
||
4 |
|
|
|
|
|
|
|
|
|
|
|
|
Skin |
|
|
|
|
4 |
|
|
|
|
|
|
|
|
|
|
|
|
|
Skin |
|
|
|
|
||||||
|
|
|
|
|
|
|
|
|
|
|
|
|
|
|
|
|
|
|
|
|
|
|
|
|
|
|
|
|
|
|
|
|
||||||||||
|
|
|
|
|
|
|
|
|
|
|
|
|
|
|
|
|
|
|
|
|
|
|
|
|
|
|
|
|
|
|
|
|
||||||||||
3 |
|
|
|
|
|
|
|
|
|
|
|
|
|
|
|
|
3 |
|
|
|
|
|
|
|
|
|
|
|
|
|
|
|
|
|
||||||||
|
|
|
|
|
|
|
|
|
|
|
|
|
|
|
|
|
|
|
|
|
|
|
|
|
|
|
|
|
|
|
|
|
||||||||||
|
|
|
|
|
|
Kidney |
|
|
|
|
|
|
|
|
|
|
|
|
|
|
|
|
|
|
|
|
|
|
|
|
|
|
|
|||||||||
2 |
|
|
|
|
|
|
|
|
|
|
|
|
|
|
|
|
2 |
|
|
|
|
|
|
|
|
Kidney |
|
|
|
|
|
|
|
|
|
|
|
|||||
|
|
|
|
|
|
|
|
|
|
|
|
|
|
|
|
|
|
|
|
|
|
|
|
|
|
|
|
|
|
|
|
|
|
|
||||||||
|
|
|
|
|
|
|
|
|
|
|
|
|
|
|
|
|
|
|
|
|
|
|
|
|
|
|
|
|
|
|
|
|
|
|
||||||||
|
|
|
|
|
|
|
|
|
|
|
|
|
|
|
|
|
|
|
|
|
|
|
|
|
|
|
|
|
|
|
|
|
|
|
|
|
|
|
|
|||
1 |
|
|
|
|
|
|
|
|
|
|
|
|
|
|
|
|
|
|
|
1 |
|
|
|
|
|
|
|
|
|
|
|
|
|
|
|
|
|
|
|
|
|
|
|
|
|
|
|
|
|
|
|
|
|
|
|
|
|
|
|
|
|
|
|
|
|
|
|
|
|
|
|
|
|
|
|
|
|
|
|
||||||
1 |
2 |
4 |
|
6 |
10 |
20 |
30 |
1 |
2 |
4 |
6 |
10 |
20 |
30 |
||||||||||||||||||||||||||||
|
|
|
|
|
X-ray dose per fraction (Gy) |
|
|
|
|
(b) |
X-ray dose per fraction (Gy) |
|
|
|
|
Figure 24.3 Comparison of relative biological effectiveness (RBE) values for mouse skin and kidney exposed to two different neutron beams: (a) d(16)-Be; (b) p(64)-Be. From Joiner (1988), with permission.
the same pattern and so must be evaluated individually in each case and for each treatment condition to determine whether high-LET radiation would result in a biological therapeutic gain. It is not true that late reactions are always worse after high-LET therapy for the same level of acute injury, but they may be in some cases unless the physical characteristics of a charged particle high-LET beam can be used to reduce the radiation dose to critical normal tissues. It should be noted that a d(16)-Be neutron beam has similar depth–dose characteristics to ortho-voltage X-rays, whereas a p(62)-Be neutron therapy beam can produce treatment plans comparable to a 4 MV X-ray beam.
Less comprehensive data are available on fractionation effects with heavy ion or proton beams. However, the loss of the fractionation dose-sparing effect, which has been described above for neutrons, is consistently less pronounced for heavy ions. However, protons must be considered – biologically, with regard to dose fractionation – as similar to photons. For both radiation qualities, heavy ions as well as protons, slight changes in RBE with dose per fraction cannot be excluded (Gerweck and Kozin, 1999). For these reasons, the number of fractions in ion radiotherapy protocols is currently close to that applied in photon therapy. For the biological reasons described above, higher doses per fraction and fewer fractions may
be possible, but supporting preclinical (in vivo) and clinical data are currently not available, and hence changes in fractionation protocols must be considered carefully.
24.3 DOSE SPECIFICATION AND ION RADIOTHERAPY PLANNING
For protons used in radiotherapy (in contrast to the extraterrestrial cosmic proton flux), a RBE factor of 1.1 is generally accepted and used routinely in clinical treatment planning programmes (Paganetti et al., 2002). Most experimental studies on the biological effectiveness for various endpoints have yielded RBE values between 1.05 and 1.25 (Tepper et al., 1977; Urano et al., 1984; ICRP, 2003). However, there might be a higher RBE value for effects within the terminal few millimetres of the beam (Gerweck and Kozin, 1999).
For heavy ions, in the last c. 2 cm of the track, an increase in RBE is seen up to values of 2–4 (Kramer et al., 2003). In the entrance channel, the biological effectiveness of heavy ions is considered only slightly higher than for protons. Complex mathematical/biophysical models are usually applied for heavy-ion treatment planning
(Kramer and Scholz, 2000; Scholz et al., 2006).

Radiotherapy with protons 335
The dose in ion beam radiotherapy is described in gray equivalents (GyE) or cobalt gray equivalents (CGE). The GyE or CGE is equal to the measured physical dose in grays multiplied by the RBE factor. Note that the term ‘equivalent dose’ in sievert (Sv) is commonly used for radiation protection purposes, with a totally different meaning from that in the present context of therapy; these should not be confused (Wambersie et al., 2006).
For radiotherapy applications, an ion beam must be extended in the lateral and longitudinal directions in order to adequately cover the clinical target volume. Depth variations can be achieved by passive absorbers (see Chapter 6, Section 6.6) or, in modern configurations, by active variation of the accelerator energy. Similarly, variations in width can be accomplished with passive devices such as scattering foils or with magnetic deflectors (pencil scanning beam). Use of beam scanning techniques will clearly result in a reduction of the normal tissue included in the high-dose volume. However, passive absorbers can increase the dose deposition outside the target volume (e.g. by neutrons that are generated at end energies 10 MeV). Furthermore, proton and heavy ion beams are much more sensitive to tissue inhomogeneities than photons. Therefore, optimum conformation of the highdose volume for minimization of normal-tissue exposure on one hand and best coverage of the planning target volume to avoid marginal recurrences on the other are highly demanding with regard to planning and technical execution of ion beam radiotherapy. Immobilization and image guidance are extremely important to limit set-up uncertainties to < 2 mm. In order to determine the necessary proton range, the density of tissue according to Hounsfield units obtained from planning computed tomography (CT) images is converted into stopping power of tissue.
The complexity of proton or heavy ion radiotherapy renders these treatments more expensive than photon radiation therapy. In a financial analysis (Goitein and Jermann, 2003), a ratio of 2.4 for cost per fraction of proton versus photon treatment was found. However, projected reductions in both construction and operational costs may reduce this ratio to 2 or even 1.5 (MacDonald et al., 2006).
24.4 RADIOTHERAPY WITH PROTONS
More than 45 000 patients have so far been treated with proton therapy worldwide. The results of clinical studies with protons have recently been subject to comprehensive reviews (MacDonald et al., 2006; Brada et al., 2007; Lodge et al., 2007; Olsen et al., 2007), and will be briefly summarized here.
Most of the early treatments were performed at physics research facilities. Because of the limited time-slots for therapy, the vast majority of patients ( 20 000) have so far been treated for uveal melanoma, where the dose is delivered within a few days. Tumour control can be achieved in more than 95 per cent of the patients, with low rates of enucleation. Analysis of the available clinical data, however, has not provided unequivocal evidence that proton therapy is superior to photon irradiation in patients with ocular melanomas (Brada et al., 2007).
Treatment of chordomas and chondrosarcomas at the base of the skull is clearly compromised by adjacent critical structures (brainstem, spinal cord, optic nerve and chiasm). The requirement of high doses in a precise location renders proton therapy attractive. In chondrosarcomas, 5-year local control rates can be as high as over 90 per cent, and are somewhat lower for chordomas, as well as for spinal and paraspinal tumours. The incidence of severe toxicities is clearly below 10 per cent. However, there are still insufficient clinical data available to formally compare toxicity after proton therapy and conventional treatment, for both chordoma and chondrosarcoma (Brada et al., 2007).
Proton treatment of glioblastoma multiforme with 90 CGE in a combined proton–photon protocol resulted in a median survival time of 20 months, but the incidence of radionecrosis was high. Protons may also be used for single fraction radiosurgery, such as for vestibular schwannoma (Weber et al., 2003) and other intracranial neoplasms.
At least 2000–3000 patients have received proton therapy for prostate cancer. The results with regard to tumour control have not been consistently better than with photons. However, fewer side-effects have been observed: standard photon techniques used over the last 30 years have resulted in severe side-effects in about 15 per cent of the patients; modern photon techniques are expected
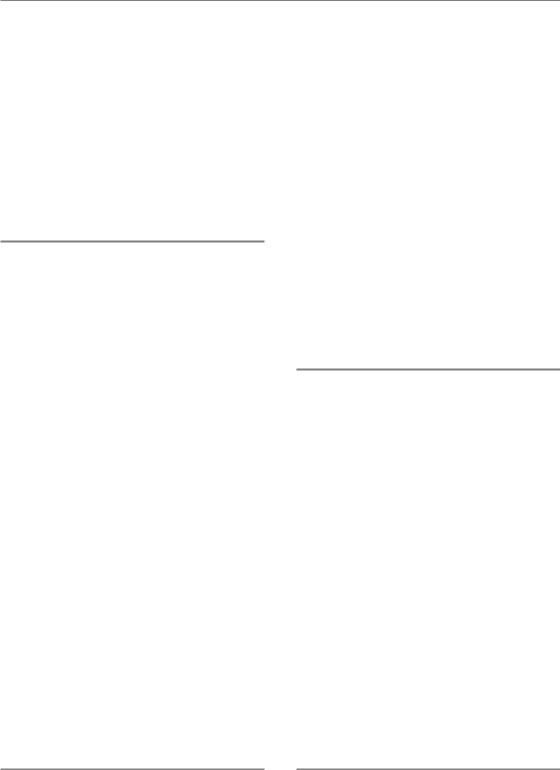
336 Protons and other ions in radiotherapy
to reduce the incidence to 1–3 per cent, and proton treatment to 1 per cent (Cox, 2007). However, a comprehensive analysis (Lodge et al., 2007) has shown an incidence of side-effects ( grade 2) of 10–30 per cent for protons and 10–40 per cent for photons.
Other malignancies being tested with proton therapy are head and neck, breast, gastrointestinal and lung tumours (MacDonald et al., 2006).
24.5 RADIOTHERAPY WITH HEAVY IONS
Heavy ion radiotherapy is more experimental than proton therapy, although some clinical data are available and have been reviewed (SchulzErtner et al., 2003; Greco and Wolden, 2007; Lodge et al., 2007). In a series of 67 patients treated with carbon ions for tumours of the base of skull (Schulz-Ertner et al., 2003) with a median tumour dose of 60 GyE, actuarial 3-year local control rates were 100 per cent for chondrosarcomas and 81 per cent for chordomas, comparable to the results with protons. For squamous cell carcinomas, the results with carbon ion therapy seem also to be similar to protons or photons. For adenoid cystic carcinomas, higher local tumour control rates ( 75 per cent) are found with carbon ions than with photons ( 50 per cent) (Lodge et al., 2007). For prostate tumours, 5-year local control rates of 95–100 per cent are reported, withgrade 2 genitourinary or gastrointestinal toxicity in 1–6 per cent of the patients.
Based on the biological properties of heavy ion beams (Section 24.2), with experimental evidence that higher doses per fraction are associated with a relatively more pronounced reduction in RBE (Fig. 24.2) for (late-responding) normal tissues compared with tumours (Denekamp et al., 1997; Ando et al., 2005), introduction of hypofractionated protocols has been suggested for clinical testing (Tsujii et al., 2004; Schulz-Ertner and Tsujii, 2007).
24.6 SECOND CANCERS
outside the planning target volume, decline the risk of secondary tumours without compromising tumour control rates. Miralbell et al. (2002) compared the potential influence of proton dose distribution with that of conformal photon treatments or intensity-modulated radiotherapy (IMRT) for the risk of second malignancies. Protons (with or without intensity modulation) clearly decreased the estimated risk compared with photon planning (with or without intensity modulation). However, these expectations have not yet been validated in experimental or clinical studies. Moreover, the advantages of protons over photons may only apply if beam scanning is available, and not for passive modulation (Schneider et al., 2002; Brenner and Hall, 2008), because of neutron generation (Section 24.3).
24.7 ION RADIOTHERAPY IN PAEDIATRIC ONCOLOGY
Radiotherapy in children must be considered more critical than in adult patients. Long-term growth deficiencies and developmental deficits must be expected. Moreover, children are at a higher risk for secondary tumours. In an analysis of children treated with radiation (Gold et al., 2004), 8.3 per cent of patients developed a second cancer, mostly within the treatment field, with a median latency of 15.5 years. The cumulative risk of second cancer was 13 per cent at 30 years. Therefore, proton or heavy ion radiotherapy may be preferred for the treatment of childhood malignancies because of the reduction in normal-tissue exposure.
Clinical reports of paediatric ion beam radiotherapy so far include various central nervous system (CNS) tumours, retinoblastoma and medulloblastoma. For medulloblastoma, local control has been in the range of that anticipated with photons but morbidity was found to be low compared with historical results.
24.8 CONCLUSIONS AND FUTURE DIRECTIONS
Theoretically, radiotherapy with protons or other ions should, by a reduction of the integral dose
The current evidence for the clinical efficacy of proton radiotherapy is predominantly based on
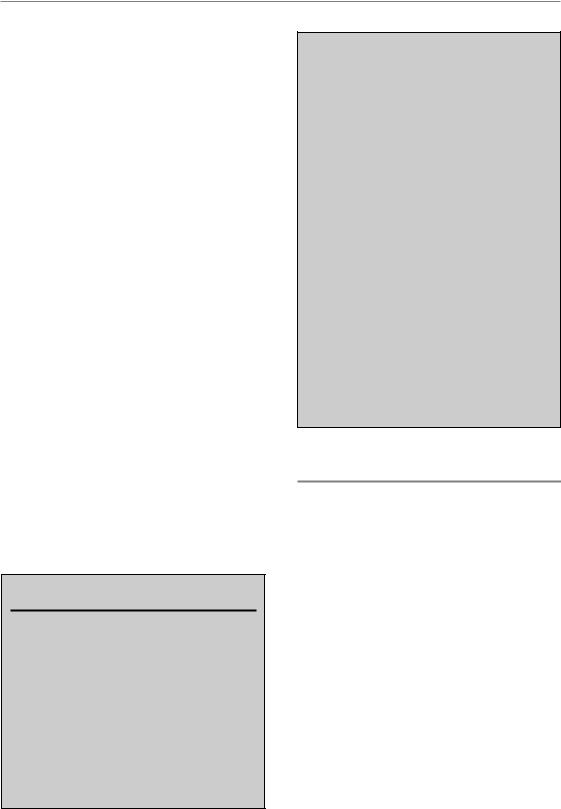
Bibliography 337
non-randomized trials (Brada et al., 2007; Lodge et al., 2007; Olsen et al., 2007). In the most common cancers, it seems unlikely that protons perform substantially better than optimized photon treatment; only a subgroup of patients with particular tumour localizations and configurations, or particular tumour biology (e.g. hypoxia) may benefit, which still must be proven in clinical trials. For ethical reasons some tumour sites (e.g. at the base of the skull), currently treated by ions at many centres, and where the dose distribution advantages are obvious, cannot be subject to randomized clinical trials. Furthermore, a reduction of rare and really late adverse effects (e.g. secondary malignancies and cardiac morbidity) is unlikely to be detected in sufficiently powered clinical investigations.
Based on prospective and retrospective studies, proton irradiation emerges as the treatment of choice for some ocular and skull base tumours. For prostate cancer, the results have been comparable to those from the best photon therapy series. However, heavy ion therapy is still in an experimental phase (Lodge et al., 2007).
For the future, one interest is in combining protons with chemotherapy (Cox, 2007), as exposure of normal tissues that may develop combined sideeffects can be reduced in proton radiotherapy. Also, the introduction of intensity-modulated proton therapy may be attractive because of its superior dose distributions compared with standard proton therapy (Miralbell et al., 2002; MacDonald et al., 2006).
Key points
1.Protons and other ions allow for dose deposition with steep dose gradients.
2.A minor fractionation effect is found for high-LET radiation (heavy ions, neutrons), while protons are comparable to photons.
3.The RBE of protons is 1.1. The RBE for heavy ions varies within the track, with low values within the entrance channel and high values of 2–4 at the Bragg peak.
4.The RBE for heavy ions and neutrons increases with decreasing dose per fraction,
and is more pronounced for late radiation responses than for acute normal-tissue and tumour effects.
5.Doses for ion irradiation are specified in CGE or GyE, which is the absorbed dose multiplied by the RBE value.
6.Proton radiotherapy has been administered for various tumour entities/localizations which require precise, highly conformal dose deposition. The clinical application is mainly based on non-controlled trials. Heavy ion therapy is even more experimental.
7.The risk for second cancers is conceptually reduced with ion treatment compared with photons, as the integral normal-tissue dose is reduced, given that active beam shaping (pencil beam scanning) is used rather than passive devices. This concept, however, has not yet been validated in clinical studies.
8.Ion therapy may be preferred for some indications in paediatric oncology, because of the reduced risk for the induction of second neoplasms.
■BIBLIOGRAPHY
Ando K, Koike S, Uzawa A et al. (2005). Biological gain of carbon-ion radiotherapy for the early response of tumor growth delay and against early response of skin reaction in mice. J Radiat Res (Tokyo) 46: 51–7.
Brada M, Pijls-Johannesma M, De Ruysscher D (2007). Proton therapy in clinical practice: current clinical evidence. J Clin Oncol 25: 965–70.
Brenner DJ, Hall EJ (2008). Secondary neutrons in clinical proton radiotherapy: a charged issue.
Radiother Oncol 86: 165–70.
Cox J (2007). Current and future status of proton-beam radiation therapy in radiation oncology. Clin Adv Hematol Oncol 5: 303–5.
Denekamp J, Waites T, Fowler JF (1997). Predicting realistic RBE values for clinically relevant radiotherapy schedules. Int J Radiat Biol 71: 681–94.
Gerweck LE, Kozin SV (1999). Relative biological effectiveness of proton beams in clinical therapy.
Radiother Oncol 50: 135–42.
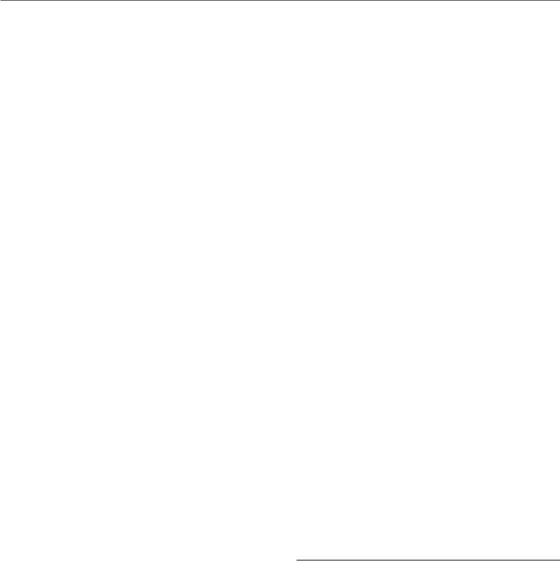
338 Protons and other ions in radiotherapy
Goitein M, Jermann M (2003). The relative costs of proton and X-ray radiation therapy. Clin Oncol 15: S37–50.
Gold DG, Neglia JP, Potish RA, Dusenbery KE (2004). Second neoplasms following megavoltage radiation for pediatric tumors. Cancer 100: 212–3.
Greco C, Wolden S (2007). Current status of radiotherapy with proton and light ion beams. Cancer 109: 1227–38.
ICRP (2003). Relative biological effectiveness (RBE), quality factor (Q), and radiation weighting factor (wR). ICRP Publication 92. Ann ICRP 33: 1–117.
Joiner MC (1988). A comparison of the effects of p(62)- Be and d(16)-Be neutrons in the mouse kidney.
Radiother Oncol 13: 211–24.
Kramer M, Scholz M (2000). Treatment planning for heavy-ion radiotherapy: calculation and optimization of biologically effective dose. Phys Med Biol 45: 3319–30.
Kramer M, Weyrather WK, Scholz M (2003). The increased biological effectiveness of heavy charged particles: from radiobiology to treatment planning.
Technol Cancer Res Treat 2: 427–36.
Lodge M, Pijls-Johannesma M, Stirk L, Munro AJ, De Ruysscher D, Jefferson T (2007). A systematic literature review of the clinical and cost-effectiveness of hadron therapy in cancer. Radiother Oncol 83: 110–22.
MacDonald SM, DeLaney TF, Loeffler JS (2006). Proton beam radiation therapy. Cancer Invest 24: 199–208.
Miralbell R, Lomax A, Cella L, Schneider U (2002). Potential reduction of the incidence of radiationinduced second cancers by using proton beams in the treatment of pediatric tumors. Int J Radiat Oncol Biol Phys 54: 824–9.
Olsen DR, Bruland OS, Frykholm G, Norderhaug IN (2007). Proton therapy – a systematic review of clinical effectiveness. Radiother Oncol 83: 123–32.
Paganetti H, Niemierko A, Ancukiewicz M et al. (2002). Relative biological effectiveness (RBE) values for proton beam therapy. Int J Radiat Oncol Biol Phys
53: 407–21.
Schneider U, Agosteo S, Pedroni E, Besserer J (2002). Secondary neutron dose during proton therapy using spot scanning. Int J Radiat Oncol Biol Phys 53: 244–51.
Scholz M, Matsufuji N, Kanai T (2006). Test of the local effect model using clinical data: tumour control probability for lung tumours after treatment with carbon ion beams. Radiat Prot Dosimetry 122: 478–9.
Schulz-Ertner D, Tsujii H (2007). Particle radiation therapy using proton and heavier ion beams. J Clin Oncol 25: 953–64.
Schulz-Ertner D, Nikoghosyan A, Thilmann C et al. (2003). Carbon ion radiotherapy for chordomas and lowgrade chondrosarcomas of the skull base. Results in 67 patients. Strahlenther Onkol 179: 598–605.
Tepper J, Verhey L, Goitein M, Suit HD (1977). In vivo determinations of RBE in a high energy modulated proton beam using normal tissue reactions and fractionated dose schedules. Int J Radiat Oncol Biol Phys 2: 1115–22.
Tsujii H, Mizoe JE, Kamada T et al. (2004). Overview of clinical experiences on carbon ion radiotherapy at NIRS. Radiother Oncol 73(Suppl 2): S41–9.
Urano M, Verhey L J, Goitein M et al. (1984). Relative biological effectiveness of modulated proton beams in various murine tissues. Int J Radiat Oncol Biol Phys 10: 509–14.
Wambersie A, Hendry JH, Andreo P et al. (2006). The RBE issues in ion-beam therapy: conclusions of a joint IAEA/ICRU working group regarding quantities and units. Radiat Prot Dosimetry 122: 463–70.
Weber DC, Chan AW, Bussiere MR et al. (2003). Proton beam radiosurgery for vestibular schwannoma: tumor control and cranial nerve toxicity.
Neurosurgery 53: 577–86.
Withers HR, Thames HD, Peters L J (1982). Biological bases for high RBE values for late effects of neutron irradiation. Int J Radiat Oncol Biol Phys 8: 2071–6.
■ FURTHER READING
Brada M, Pijls-Johannesma M, De Ruysscher D (2007). Proton therapy in clinical practice: current clinical evidence. J Clin Oncol 25: 965–70.
Brenner DJ, Hall EJ (2008). Secondary neutrons in clinical proton radiotherapy: a charged issue.
Radiother Oncol 86: 165–70.
Lodge M, Pijls-Johannesma M, Stirk L, Munro AJ, De Ruysscher D, Jefferson T (2007). A systematic literature review of the clinical and cost-effectiveness of hadron therapy in cancer. Radiother Oncol 83: 110–22.
MacDonald SM, DeLaney TF, Loeffler JS (2006). Proton beam radiation therapy. Cancer Invest 24: 199–208.
Olsen DR, Bruland OS, Frykholm G, Norderhaug IN (2007). Proton therapy – a systematic review of clinical effectiveness. Radiother Oncol 83: 123–32.