
- •Contents
- •Preface
- •1 Introduction: the significance of radiobiology and radiotherapy for cancer treatment
- •2 Irradiation-induced damage and the DNA damage response
- •3 Cell death after irradiation: how, when and why cells die
- •4 Quantifying cell kill and cell survival
- •5 Dose–response relationships in radiotherapy
- •6 Linear energy transfer and relative biological effectiveness
- •7 Tumour growth and response to radiation
- •8 Fractionation: the linear-quadratic approach
- •9 The linear-quadratic approach in clinical practice
- •10 Modified fractionation
- •11 Time factors in normal-tissue responses to irradiation
- •12 The dose-rate effect
- •13 Pathogenesis of normal-tissue side-effects
- •14 The volume effect in radiotherapy
- •15 The oxygen effect and fractionated radiotherapy
- •16 The tumour microenvironment and cellular hypoxia responses
- •17 Therapeutic approaches to tumour hypoxia
- •18 Combined radiotherapy and chemotherapy
- •19 Retreatment tolerance of normal tissues
- •20 Molecular image-guided radiotherapy with positron emission tomography
- •21 Molecular-targeted agents for enhancing tumour response
- •22 Biological response modifiers: normal tissues
- •23 Molecular targeting and patient individualization
- •24 Protons and other ions in radiotherapy
- •25 Second cancers after radiotherapy
- •Glossary of terms in radiation biology
- •Index
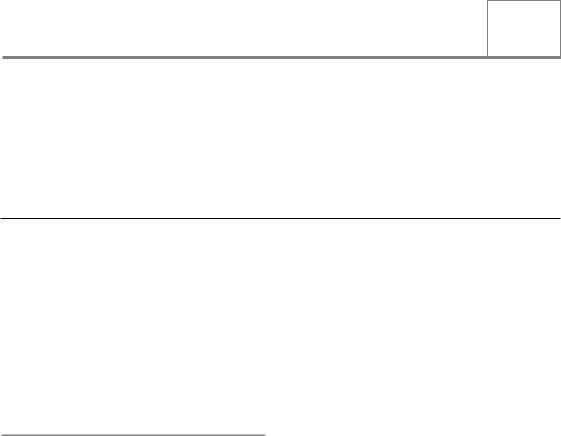
20
Molecular image-guided radiotherapy with positron emission tomography
VINCENT GRÉGOIRE, KARIN HAUSTERMANS AND JOHN LEE
20.1 |
Introduction: molecular imaging and |
|
20.5 Theragnostic imaging for radiation |
|
|
its potential use in modern radiotherapy |
271 |
oncology |
282 |
20.2 |
Image acquisition and reconstruction |
|
Key points |
283 |
|
with PET |
273 |
Bibliography |
283 |
20.3 |
PET image segmentation |
274 |
Further reading |
286 |
20.4 |
Using PET images for treatment planning |
276 |
|
|
|
|
|
|
|
20.1 INTRODUCTION: MOLECULAR IMAGING AND ITS POTENTIAL USE IN MODERN RADIOTHERAPY
Molecular imaging, also referred to as biological imaging or functional imaging, is the use of noninvasive imaging techniques that enable the visualization of various biological pathways and physiological characteristics of tumours and/or normal tissues. In short, it mainly refers (but not only) to positron emission tomography (PET) and functional magnetic resonance imaging (fMRI). In clinical oncology, molecular imaging offers a unique opportunity to allow an earlier diagnosis and staging of the disease, to contribute to the selection and delineation of the optimal target volumes for radiotherapy and, to a lesser extent, for surgery, to evaluate the response early in the treatment or after its completion, and to help in the early detection of recurrence (Fig. 20.1). From the viewpoint of experimental oncology, molecular imaging may also facilitate and speed up the process of drug development by allowing faster and cheaper pharmacokinetic and biodistribution studies.
For target volume selection and delineation, anatomic imaging modalities such as computed tomography (CT), and to a lesser extent magnetic resonance imaging (MRI), remain the most widely used modalities. Over the last few years, however, the use of molecular imaging and in particular the use of positron-labelled fluorodeoxyglucose (FDG-PET) has become increasingly widespread. Provided that appropriate tracers are used, molecular imaging with PET enables the visualization of the various molecular pathways in tumours, including metabolism, proliferation, oxygen delivery and consumption, and receptor or gene expression, all of which may be important in the response to ionizing radiation.
The goal of radiotherapy treatment planning is to select and delineate target volumes (and organs at risk) based on all the available diagnostic information and on the knowledge of the physiology of the disease (i.e. the probability of local and nodal infiltration). This is done in part by using various imaging modalities, which depict more or less accurately the true tumour extent. The difficulty with using imaging modalities is that none of them has a sensitivity (no false negatives) or a
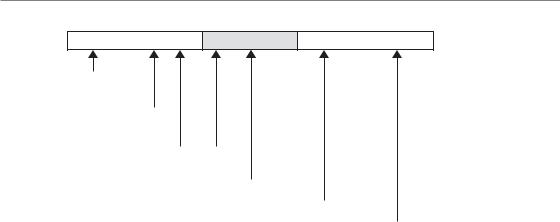
272 Molecular image-guided radiotherapy with positron emission tomography
S/RxTh/CH
Work-up-staging prognostic evalution
GTV/CTV
Selection and delineation
Functional image-guided IMRT
Early response evalution
Final response evalution
Early detection of recurrence
Figure 20.1 Relative timescale illustrating the various opportunities for the use of biological imaging in oncology. S/Rx Th/Ch, surgery and/or radiotherapy and/or chemotherapy.
specificity (no false positives) of 100 per cent. Thus, false negatives and false positives for depicting neoplastic processes occur.
How the sensitivity and specificity of a particular imaging modality influences the radiotherapy planning process depends on the underlying objective of the treatment. If, for a particular disease, the objective is to avoid missing tumour at any cost, a highly sensitive approach needs to be selected. This will give a lower specificity, resulting in inclusion of non-neoplastic tissue into the target volume. However, this approach reduces the likelihood that neoplastic cells are missed. If, on the other hand, the aim is to avoid including non-neoplastic cells into the target volume to protect normal tissue, a highly specific approach needs to be selected. However, this reduces the sensitivity of the approach and increases the risk of missing tumour cells. Therefore, the additional use of PET in treatment planning, and of FDG-PET in particular, has assumed increasing importance to the point where many radiation oncologists now believe that target volume selection and delineation cannot be adequately performed without the use of PET.
When incorporating PET into treatment planning, its sensitivity and specificity should be compared with CT and with pathological verification of tumour extent from surgical sampling, if available. The potential impact of PET on treatment planning needs to be determined. For example, if an additional lymph node is visualized with a new imaging modality known to be more specific than
the standard modality, it might be legitimate to increase the target volume(s) beyond what would have been given using a standard procedure; conversely, if fewer nodes are visualized with a new imaging modality known to be more sensitive than the standard modality, it might be legitimate to decrease the target volume(s) below what would have been given using a standard procedure.
Table 20.1 summarizes data on the specificity and sensitivity of FDG-PET and CT (or MRI) for lymph node staging in lung cancer, head and neck cancer, cervical cancer, oesophageal cancer and colorectal cancer, comparing with surgical lymph node sampling as the gold standard. In head and neck tumours, CT and FDG-PET performed with a comparable diagnostic accuracy. A potentially interesting use of FDG-PET is in the staging of node-negative patients (as assessed by other imaging modalities) where the issue could be to avoid treating the neck nodes if an FDG-PET examination also turns out to be negative. However, in the node-negative neck, the sensitivity of FDG-PET, compared with the examination of the pathology specimen after a neck node dissection, is only around 70 per cent (Stuckensen et al., 2000). This is not surprising in light of the fact that in nodenegative patients who underwent a prophylactic neck node dissection, microscopic nodal infiltration could nevertheless be observed in up to 30 per cent of cases (Shah, 1990). Thus the rather low signal-to-background ratio of FDG and the limited spatial resolution of the cameras currently
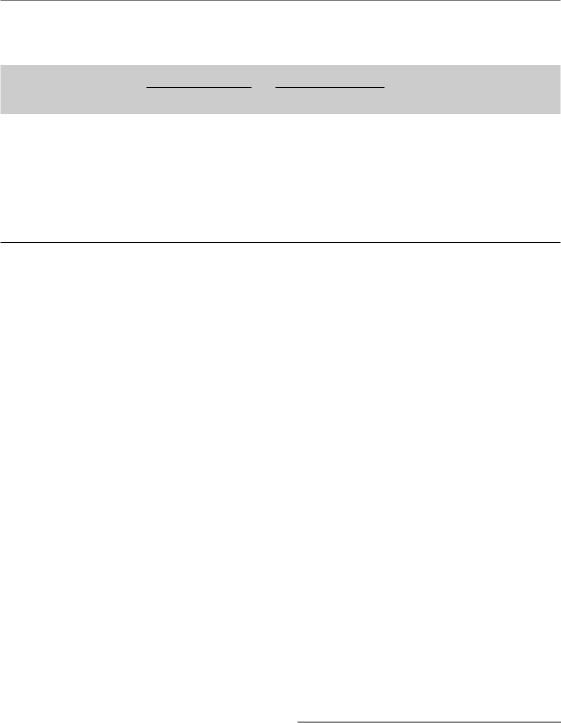
Image acquisition and reconstruction with PET 273
Table 20.1 Comparison between computed tomography (CT) (or magnetic resonance imaging, MRI) and fluorodeoxyglucose positron emission tomography (FDG-PET) for nodal staging
Sensitivity (%) Specificity (%)
Site |
CT |
FDG-PET |
CT |
FDG-PET |
Reference |
Head and neck cancer |
36–86 |
50–96 |
56–100 |
88–100 |
Menda and Graham (2005) |
Non-small cell lung cancer |
45 |
80–90 |
85 |
85–100 |
Birim et al. (2005) |
Cervix carcinoma |
57–731 |
75–91 |
83–1001 |
92–100 |
Rose et al. (1999), Sugawara |
|
|
|
|
|
et al. (1999), Reinhardt |
|
|
|
|
|
et al. (2001) |
Oesophageal cancer |
11–87 |
30–78 |
28–99 |
86–98 |
van Westreenen et al. (2004) |
Colorectal carcinoma |
29 |
29 |
96 |
85 |
Abdel-Nabi et al. (1998) |
1CT or MRI.
preclude the detection of microscopic disease with PET and, therefore, compared with anatomic imaging modalities such as CT and MRI, it is unlikely that FDG-PET will be of any value in selecting nodal target volumes in the neck.
Conversely, when evaluating the added value of FDG-PET in non-small cell lung cancer (NSCLC), the opposite is true. The sensitivity for staging of lymph nodes in lung cancer is significantly higher for FDG-PET than for CT. This implies that a negative PET scan could result in substantially reduced target volumes and permit focusing on the primary tumour.
In esophageal cancer, the sensitivity of FDGPET is similar to that of CT. Conversely, FDG-PET is very specific for the staging of lymph nodes outside of the mediastinum (e.g. supra-clavicular or coeliac lymph nodes). If such lymph nodes are detected with FDG-PET, it is legitimate to enlarge the selection (thus the delineation) of the target volume (Vrieze et al., 2004).
In para-aortic lymph nodes of patients with cervix carcinoma, although based on a limited number of patients, FDG-PET is also reported to be much more specific than CT or MRI, thus supporting the inclusion of these nodes in case of positive PET findings. It has also been shown that FDG-PET positive findings in the para-aortic region were a predictor of overall survival after treatment with radiotherapy and chemotherapy (Grigsby et al., 2001).
In colorectal carcinoma, FGD-PET was slightly more specific than CT for the detection of lymph
node infiltration (Table 20.1), but owing to the quasi-systematic use of preoperative chemoradiotherapy on the whole pelvis, the value of this finding is unclear. However, it might be useful to support a change in the therapeutic management in cases of a positive lymph node outside of the pelvic cavity.
The data in Table 20.1 were obtained with PET cameras, whereas centres are increasingly being equipped with dual PET/CT systems. Few systematic comparisons between the diagnostic accuracy of standalone PET and integrated PET/CT have been performed. Overall, diagnostic accuracy might be improved by the use of dual PET/CT cameras (Bar-Shalom et al., 2003; Antoch et al., 2004). It is, however, interesting to note that, although logistically more demanding, the performance of the side-by-side PET and CT comparison was almost as good as the dual cameras (Antoch et al., 2004). Whatever the improved diagnostic accuracy of combined PET and CT examinations, the results of more extensive comparisons between PET/CT and PET alone have to be awaited before definitive conclusions can be drawn.
20.2 IMAGE ACQUISITION AND RECONSTRUCTION WITH PET
In oncology, PET has been used routinely as a diagnostic tool for detection of lesions. By contrast, volume delineation on PET images appears as a relatively recent trend in radiotherapy. In comparison with simple diagnosis, volume delineation
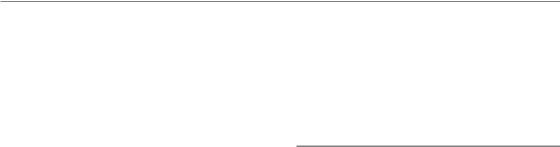
274 Molecular image-guided radiotherapy with positron emission tomography
requires greater care in acquisition, reconstruction and processing of PET images, in order to minimize the uncertainty on tumour boundaries and achieve reasonable volume accuracy.
First, it is useful to recall some inherent limitations of PET (Tarantola et al., 2003). For many physical reasons (e.g. optimal size of detector chosen for efficiency reasons, positron energy, etc.) whose discussion is beyond the scope of this chapter, the resolution of PET images is lower than images from CT or MR: typical PET cameras have about four times lower resolution than modern CT scans, which explains the blurry aspect of PET images. Also, because PET is an emission modality, its images suffer from a high noise level. The injected dose of the tracer must be limited for obvious radioprotective reasons. Moreover, many positron disintegrations occur outside the field of view of the PET camera where they do not contribute to the image but can add additional noise (scattered events). Both the low resolution and the high noise level must be taken into account when selecting suitable acquisition protocols and reconstruction procedures. In summary, the best sensitivity is achieved with three-dimensional (3D) acquisition; this also allows a good trade-off between tracer-injected dose, acquisition duration and patient comfort. Regarding reconstruction protocols, iterative algorithms such as Ordered Subsets Expectation Maximization (OSEM) is preferred, especially for accurate volumetric assessment. This reconstruction method is, however, quite slow and may not be suitable for routine diagnostic PET acquisition.
After the images have been reconstructed, further enhancements can be achieved by filtering and deblurring. Filtering aims at further reducing the noise to enhance the gradient between the background activity and the signal of interest. When image quality is essential, as it is for automatic segmentation, an anisotropic diffusion filter or bilateral filter is preferred to the typical Gaussian filter (Nagayoshi et al., 2005). A typical example of what image filtering does is shown in Fig. 20.2. Image deblurring aims at restoring sharp edges between voxels of low activity and those of high activity. Such methods require an accurate knowledge of the resolution characteristics of the PET camera. In addition, because of the
longer computation time required by those techniques, small objects (with a size approximating the resolution of the camera) cannot be correctly segmented.
20.3 PET IMAGE SEGMENTATION
The accurate determination of the volume and shape of the tumour from PET images remains a challenge and an incompletely resolved issue. Although most of the reports on the segmentation of PET images have dealt with non-small cell lung cancer, various methods have been already tested to determine the outline of FDG-positive tissue in patients with head and neck squamous carcinoma (HNSSC) (Erdi et al., 1997).
The easiest and simplest method consists of visual interpretation of the PET images and definition of the tumour contours by an experienced nuclear medicine physician or a radiation oncologist (Ciernik et al., 2003). However, this method appears highly debatable. First, the threshold level of the PET image, which depends on the display windowing, strongly influences the visual assessment of the tumour boundaries. Moreover, the visual delineation of objects is a subjective approach that will necessarily lead to substantial intraand interobserver variability.
Within this framework, the development of objective and reproducible methods for segmenting PET images has become crucial. The simplest method relies on the choice of a fixed threshold of activity (i.e. a given percentage of the maximal activity within the tumour, for distinguishing between malignant and surrounding normal tissues). Using a fixed threshold of 50 per cent of the maximal activity to automatically segment a primary tumour of the head and neck region, tumour volumes delineated from PET images with FDG were larger than those delineated with CT in 25 per cent of the cases (Paulino et al., 2005). However, results from this study have to be viewed with caution since the relevance of an arbitrary fixed threshold appears questionable. Indeed, it has been shown that the threshold required to match macroscopic laryngectomy specimens used as a ‘gold standard’ varied from one specimen to another between 36 per cent and
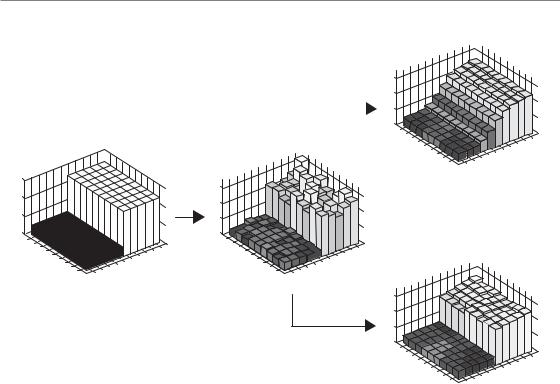
PET image segmentation 275
Filtered data
|
6 |
|
Usual filter |
4 |
|
2 |
||
|
||
|
0 |
|
|
|
Noise-free |
Noisy data |
1 2 3 4 |
5 6 7 8 |
|
|
3 4 5 6 7 |
8 9 10 |
data |
|
9 |
1 2 |
|
|||
|
|
|
|
|
|
||
6 |
6 |
|
|
|
|
|
|
|
|
|
|
|
|
|
|
4 |
4 |
|
|
|
|
|
|
|
|
|
|
|
|
|
|
2 |
2 |
|
|
|
|
|
|
|
|
|
|
|
|
|
|
0 |
0 |
|
|
|
|
|
|
1 2 |
3 4 |
|
|
|
|
|
|
|
|
9 10 |
1 2 |
3 4 |
|
|
|
|
|
|
|
|
|
9 10 |
|
|
|
Filtered data |
|
|||
|
5 |
6 |
|
|
|
|
6 |
7 8 |
|
5 |
6 |
|
|
|
|
|
6 |
7 8 |
|
|
|
|
||||||||
|
|
7 8 |
|
|
3 4 5 |
|
|
|
7 8 |
|
|
3 4 |
5 |
|
|
|
|
|
|
|
|
|
||||||||
|
|
|
|
|
|
|
|
|
|
|
|
|
|
|
|
|
|
|
|
|
|
|||||||||
|
|
|
|
9 |
1 2 |
|
|
|
|
|
|
|
9 |
1 2 |
|
|
|
|
|
|
|
|
|
|
|
|||||
|
|
|
|
|
|
|
|
|
|
|
|
|
|
|
|
|
|
|
|
|
|
|
|
|
|
|
||||
|
|
|
|
|
|
|
|
|
|
|
|
|
|
|
|
|
|
|
|
|
|
6 |
|
|
|
|
|
|
|
|
|
|
|
|
|
|
|
|
|
|
|
|
|
|
|
|
|
|
|
|
|
|
4 |
|
|
|
|
|
|
|
|
|
|
|
|
|
|
|
|
|
|
|
|
|
|
|
|
|
|
|
|
|
|
2 |
|
|
|
|
|
|
|
|
|
|
|
|
|
|
|
|
|
|
|
|
|
|
|
|
|
Edge- |
|
|
|
0 |
|
|
|
|
|
|
|
|
|
|
|
|
|
|
|
|
|
|
|
|
|
|
|
|
|
|
preserving |
1 2 |
3 4 5 |
|
|
|
|
|
|
8 9 10 |
||||
|
|
|
|
|
|
|
|
|
|
|
|
|
|
|
|
|
filter |
|
|
|
|
6 |
7 8 |
|
|
3 4 5 |
6 7 |
|||
|
|
|
|
|
|
|
|
|
|
|
|
|
|
|
|
|
|
|
|
|
|
|
|
|
|
|
||||
|
|
|
|
|
|
|
|
|
|
|
|
|
|
|
|
|
|
|
|
|
|
|
|
|
9 |
1 2 |
|
|
||
|
|
|
|
|
|
|
|
|
|
|
|
|
|
|
|
|
|
|
|
|
|
|
|
|
|
|
|
|
Figure 20.2 The principle of edge-preserving filtering. An ‘ideal’ positron emission tomography (PET) image consists of two regions, one with a low activity and another with a higher activity (on left). The activity is depicted with bars of varying height. ‘Real’ PET scanners do not yield noise-free images, and a simulation of a noisy ‘real’ image is depicted in the centre. From there, two different filters have been used. The top right image is obtained with a usual Gaussian filter, whereas the bottom right image results from the application of an edge-preserving filter. This ‘real’ image is much closer to the ‘ideal’ image.
73 per cent of the maximal activity (Gregoire et al., 2005). Reinforced by the absence of validation studies, these data clearly illustrate that methods based on fixed thresholds are not adequate for accurately segmenting tumours from PET images.
The use of an adaptive threshold is an elegant option that could eliminate the drawbacks of methods described above. Adaptive thresholding relies on a model that determines the appropriate threshold of activity according to the signal-to- background ratio. This method has been shown to be accurate for segmenting PET images in a series of pharyngo-laryngeal tumours (Daisne et al., 2004). Although validated as a reliable segmentation method, it nevertheless presents some drawbacks. For example, it is unclear whether the method is valid across different centres as it depends on so many centre-dependent parameters such as the
camera and its point spread function (PSF), and the reconstruction and filtering method. Also, this method is not ideal for images with low signal to background ratios, such as encountered in peritumoural inflammation induced by radiotherapy or in undifferentiated tumours.
Gradient-based segmentation methods, which are used for CT, cannot be directly employed for PET because of its poor resolution. However, image restoration tools, such as edge-preserving filters and deblurring algorithms (see Section 20.2), partly overcome the problems of blur and noise and may enable gradient-based segmentation techniques to be adopted. Preliminary experiments have shown encouraging results with segmentation of phantom objects and head and neck tumours based on the combination of watershed transform and hierarchical cluster analyses
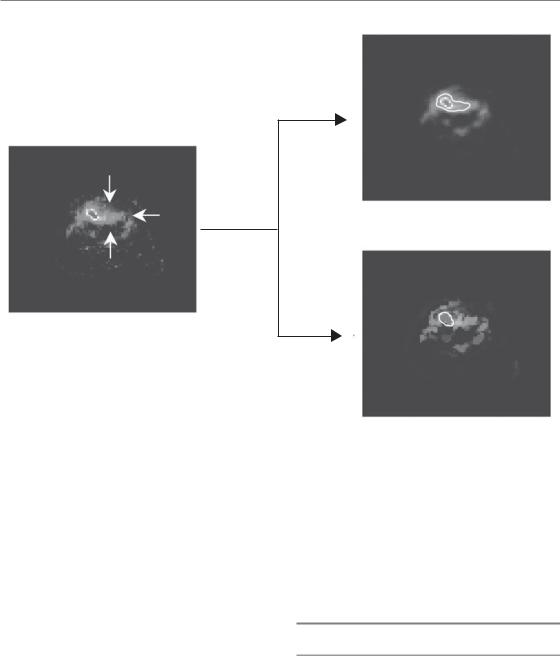
276 Molecular image-guided radiotherapy with positron emission tomography
Threshold-based
Raw image
Image segmentation
Gradient-based
Figure 20.3 Comparison between a threshold-based and a gradient-based method for the automatic segmentation of a head and neck tumour during radiotherapy. The image is intrinsically noisier because of peritumoural radiationinduced mucositis. The gradient-based method led to a more specific tumour segmentation. See colour plate section for full colour image.
(Geets et al., 2007a). The main advantage of this approach is that it is purely data-driven; no underlying model or calibration curve is necessary, except the knowledge of the scanner PSF. Consequently, both the applicability of the method and its spread could be increased since it could still yield a reasonable segmentation in difficult cases (e.g. an image with low signal-to- background ratio), where threshold-based methods usually fail. A typical example is the use of FDGPET during radiotherapy (Fig. 20.3). The combination of radio-induced mucositis, which increases the background signal, together with the reduction in tumour uptake secondary to the treatment response, leads to a drastic decrease in signal-to- background ratio. In this context, resolving the residual tumour from the surrounding inflammatory area requires powerful segmentation methods
that are able to detect gradient-intensity crests of low magnitude and/or delayed imaging acquisition.
20.4 USING PET IMAGES FOR TREATMENT PLANNING
Brain tumours
Unlike other tissues, the brain almost exclusively metabolizes glucose to meet its energy demands. Consequently, the accumulation of FDG in normal brain tissue is very high and this limits the use of FDG-PET for brain tumour imaging. Thus, compared with MRI, FDG-PET provides additional information for radiotherapy planning only in a minority of cases. This is because of the small difference in contrast between viable tumour and
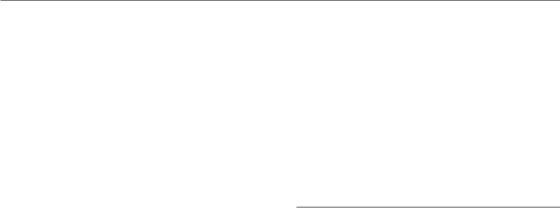
Using PET images for treatment planning 277
normal white matter even for many high-grade tumours. Because uptake of 11C-labelled methionine ([11C]-MET) in normal brain parenchyma is low, MET-PET is superior to FDG-PET in the assessment of tumour dimensions. The most important radiolabelled amino acid tracers used for brain imaging are 11C-labelled MET, 132I-labelled α-methyl-tyrosine (IMT) and 18F-labelled O-(2) fluoroethyl-L-tyrosine (FET). The short half-life of [11C]-MET limits its clinical usefulness to centres with on-site cyclotrons. However, FET is an attractive alternative, as it was shown that MET-PET and FET-PET were equal in their ability to diagnose vital glioma tumour tissue (Weber et al., 2000).
The uptake of MET is correlated to prognosis and higher MET uptake has been seen in grade III or IV gliomas, compared with low-grade gliomas. Moreover, oligodendrogliomas tend to show a higher uptake of MET than astrocytomas, which is probably linked to oligodendroglial cellular differentiation (Herholz et al., 1998). However, a number of studies have provided controversial findings on the accuracy of tracer uptake in determining tumour grade (e.g. Schmidt et al., 2001).
There are only limited data available on the usefulness of MET-PET in radiotherapy treatment planning. Several studies have shown that the margins of tumours, as assessed by PET with amino acid tracers, are frequently wider than those assessed by MRI or CT (Jacobs et al., 2002). MET-PET detects solid parts of brain tumours as well as the infiltration area with high sensitivity (87 per cent) and specificity (87 per cent) and this phenomenon can be even more pronounced in low-grade tumours and diffuse gliomatosis because of their lack of contrast enhancement on MRI (Kracht et al., 2004). Sensitivity and specificity of MET-PET in differentiating between non-tumour tissue and low-grade gliomas was 76 per cent and 87 per cent, respectively (Herholz et al., 1998). In 14 patients of whom 13 had low-grade astrocytomas, MET-PET was helpful in delineating the gross tumour volume (GTV) in 27 per cent, whereas PET findings either correlated with MRI (46 per cent) or were less distinctive in 27 per cent (Nuutinen et al., 2000). In meningiomas, difficulties in tumour delineation may occur as these tumours frequently infiltrate and because contrast enhancement in the normal tissues may be comparable to that of the tumour. Meningioma borders
can be more accurately defined on the basis of METPET/CT (Grosu et al., 2003; Sweeney et al., 2003). In conclusion, MET-PET can be helpful in delineating brain tumours for radiotherapy planning but more studies, especially with the alternative FET, tracer are needed to recommend its use in daily radiation treatment planning (Grosu et al., 2005).
Head and neck squamous cell carcinoma
As discussed above, the value of FDG-PET for the selection of target volumes in the head and neck area has yet to be demonstrated. Indeed, its sensitivity and specificity for the assessment of head and neck node infiltration does not differ significantly from that of CT or MRI (Table 20.1). However a study in 20 patients with mostly locally advanced disease demonstrated an increase in sensitivity with the use of a hybrid PET/CT compared with CT alone and showed that PET/CTbased radiation treatment would have significantly changed the dose distribution (Schwartz et al., 2005). These findings need to be confirmed prospectively in larger study populations before it can be implemented into routine use.
In contrast, FDG-PET has been shown to be of value for the delineation of the primary tumour GTV by comparing 3D registration of CT, MRI and FDG-PET images of oropharyngeal, hypopharyngeal and laryngeal squamous cell carcinomas (Daisne et al., 2004). In a subset of laryngeal tumours, the imaging modalities were also registered with the actual surgical specimen taken as a ‘gold standard’. It was found that MRI did not provide any added value to CT, either in terms of volumetric GTV assessment or in terms of reduced interobserver variability (Geets et al., 2005). In contrast, FDG-PET demonstrated higher accuracy in delineating GTV with a statistically significant reduction in the target volumes. All three imaging modalities, however, failed to visualize the extent of superficial tumour, illustrating their limitations in spatial resolution. Interestingly, the differences observed between CT and FDG-PET for the GTV delineation translated into significant differences in clinical target volume (CTV) and planning target volume (PTV) delineation. When comparative
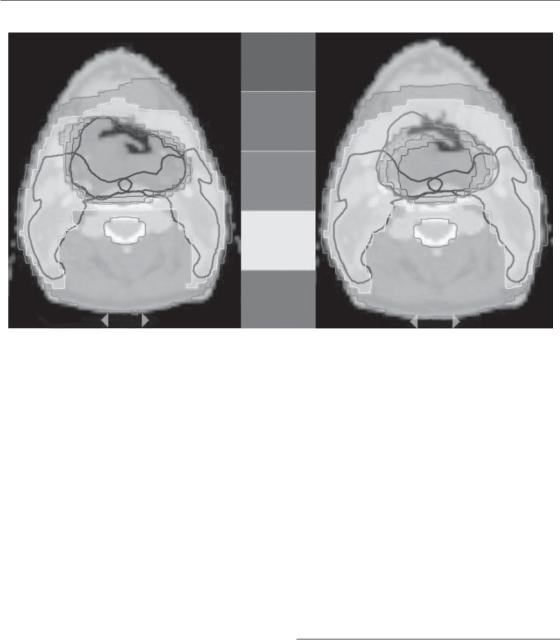
278 Molecular image-guided radiotherapy with positron emission tomography
A
A
65.55
62
59
52.44
P 40 P
152 |
152 |
|
Figure 20.4 Patient with a T4-N0-M0 squamous cell carcinoma of the hypopharynx. The patient was treated using a simultaneous integrated boost approach delivering a dose of 55.5 Gy (30 fractions of 1.85 Gy) to the prophylactic planning target volume (PTV; delineated in dark blue) and a dose of 69 Gy (30 fractions of 2.3 Gy) to the therapeutic PTV (delineated in dark green). Comparison between a pretreatment computed tomography (CT)-based plan (left) and adaptive fluorodeoxyglucose positron emission tomography (FDG-PET)-based plan (right). On both plans, only the CTbased PTVs are depicted. A FDG-PET examination was performed before treatment and at 16 Gy, 24 Gy, 34 Gy and 44 Gy. The dose distribution was adapted to the progressive reduction of the FDG-PET gross tumour volume (GTV). See colour plate section for full colour image.
3D-conformal radiotherapy (CRT) plans were made, FDG-PET-based plans were more conformal than the CT-based plans and reductions in the isodose volumes with subsequent reductions in the dose to the surrounding normal tissues were observed in the PET-based plans (Geets et al., 2006). This could have important consequences as it paves the way for possible dose escalation to the target volumes; in a proof-of-concept study on 15 patients with head and neck tumours, it was shown that an increase in dose per fraction (to 2.5 Gy and 3 Gy per fraction) could be safely delivered to the FDG-PET-based PTV during part of the treatment (Vanderstraeten et al., 2006).
During a course of fractionated radiotherapy, it is anticipated that both anatomical and functional tumour changes will occur. Re-assessment of the tumour during radiotherapy with subsequent adaptation of the plan might thus allow a much tighter dose distribution to the target volumes
with consequent decrease of the total irradiated volume. In the hypopharynx, it has been shown (Geets et al., 2007b) that the GTV progressively decreases during radiotherapy and that adaptive treatment could lead to a significant reduction in the high-dose volume in some cases (Fig. 20.4).
Non-small cell lung cancer (NSCLC)
Fluorodeoxyglucose-PET has a higher sensitivity/ specificity for nodal staging than CT and might thus alter the GTV either by detecting unnoticed metastatic lymph nodes or by downstaging a CT false-positive mediastinal nodal station. Because of the particularly high sensitivity, the second situation is more frequent (Table 20.1). In a series of 44 patients, it was shown that FDG-
PET altered the stage of the disease in 11 patients
(25 per cent) by down-staging 10 of these patients
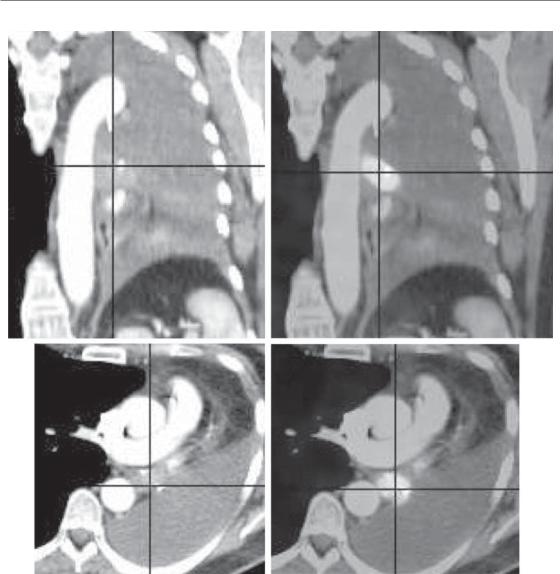
Using PET images for treatment planning 279
(a) |
|
(b) |
|
|
|
(c) |
|
(d) |
|
|
|
Figure 20.5 This example illustrates the role of fluorodeoxyglucose positron emission tomography (FDG-PET) for delineating the volume of a lung cancer. The images represent saggital (a) and axial (c) slices of a patient with a lung cancer located in the left hilar region, with retro-obstructive atelectasis of the entire left lung, associated with a major pleural effusion. The metabolic information provided by FDG-PET (b, d) shows that the tumour tissue is strictly located to the hilum. The delineation of the tumour margins is easier and more accurate with the help of FDG-PET, allowing for a significant modification of the target volume. See colour plate section for full colour image.
(De Ruysscher et al., 2005b). As a consequence, the GTV based on FDG-PET was, on average, smaller than the GTV defined on CT. In a simulation study, it has been shown that for the same expected toxicity to lungs, spinal cord and oesophagus, the dose to the tumour could be increased by 25 per cent, resulting in a potentially
higher tumour control probability of 24 per cent for PET-CT planning compared with 6.3 per cent for CT alone (De Ruysscher et al., 2005a). In addition to better detection of true positive lymph nodes, FDG-PET further alters the definition of GTV by discriminating tumour tissue from atelectasis or necrosis (Fig. 20.5). Other studies

280 Molecular image-guided radiotherapy with positron emission tomography
have reported that FDG-PET alters the GTV in 22–62 per cent of the patients (Bachaud et al., 2005) and PET, especially PET-CT, imaging has been shown to significantly reduce the interobserver variability, as well as the intraobserver variability (Ashamalla et al., 2005; Fox et al., 2005). In a modelling study, it was reported in 21 patients with N2–N3 NSCLC that the use of PETCT for radiotherapy planning resulted in a lower radiation exposure of the oesophagus and the lungs, allowing a significant dose escalation to the tumour (van der Wel et al., 2005).
To date, only a few studies have prospectively included PET with FDG in radiotherapy planning and actually addressed its impact on local tumour control and survival. Selective mediastinal node irradiation based on PET with FDG has yielded a low rate of isolated nodal failure, suggesting that reducing the target volume does not result in worse local control (De Ruysscher et al., 2005b).
Some issues related to the use of PET in lung cancer radiotherapy remain unresolved. First, tumour delineation/contouring by PET is still unsatisfactory, as discussed in Section 20.3. The appropriate activity threshold to be used to automatically delineate the tumour contours varies with the size of the tumour and the tumour- to-background ratio (Yaremko et al., 2005). It has been suggested that the area of lower uptake (which has been named the ‘anatomic biologic halo’) immediately surrounding the most metabolic part of the tumour be included in the GTV defined from PET (Ashamalla et al., 2005). Including this ‘halo’ resulted in a better dose coverage of the PTVs. Standardization is needed, since the use of different delineation techniques for FDG leads to different GTVs (Nestle et al., 2005).
The second methodological issue, of particular importance in lung cancer radiation therapy, is tumour motion during PET imaging and radiotherapy. The PET images are usually acquired during free breathing. Usual emission scan duration for PET is 5–10 min per bed position for conventional PET. For PET/CT, the short CT scan is used for attenuation correction. To increase the accuracy of tumour volume delineation, respiratory gating techniques should be implemented
(Boucher et al., 2004).
Esophageal tumour
As already discussed, FDG-PET is particularly specific for lymph node detection outside of the mediastinum, which might help to optimize the radiation target volume (Table 20.1). In an analysis of the additional value of FDG-PET for optimization of the CTV in 30 patients with advanced oesophageal cancer (Vrieze et al., 2004), discordances between conventional staging modalities, including CT and oesophageal endoscopic ultrasound (EUS), for the detection of lymph node involvement were found in 14 out of 30 patients (47 per cent). In eight patients, the involved lymph nodes were detected only on CT/EUS, which would have led to a decrease in the CTV in three of them if PET alone had been used. In contrast, PET with FDG was the only detector of lymph node involvement in six patients, resulting in a possible larger CTV in three of these patients (10 per cent). The authors concluded that the high specificity of FDG-PET for lymph node detection justifies its use for treatment volume adaptation in case of positive findings, while the low sensitivity of FDGPET (i.e. false-negative lymph nodes) would give an erroneous reduction of the CTV. Whether the role of FDG-PET in esophageal treatment planning will lead to a therapeutic gain without increasing the toxicity remains unanswered.
Another study evaluated the impact of CT and FDG-PET in conformal radiotherapy in 34 patients with oesophageal carcinoma referred for radical chemo-radiation (Moureau-Zabotto et al., 2005). After manual delineation of the GTV on both modalities, CT and PET were co-registered. Image fusion (GTV-PET was used as overlay to GTV-CT) resulted in a reduction of the GTV in 12 patients (25 per cent) and an increase in seven patients (21 per cent). Modification of the GTV affected the PTV in 18 patients and affected the percentage of lung volume receiving more than 20 Gy in 25 patients (74 per cent), with a dose reduction in 12 patients and a dose increase in 13 patients. A similar study was performed with an integrated PET/CT scanner (Leong et al., 2004). Here, the GTV was enlarged in 9 out of 10 (90 per cent) patients by a median volume of 22 per cent (range 3–100 per cent) when PET with FDG information was added to the CT-based GTV. In three patients, the
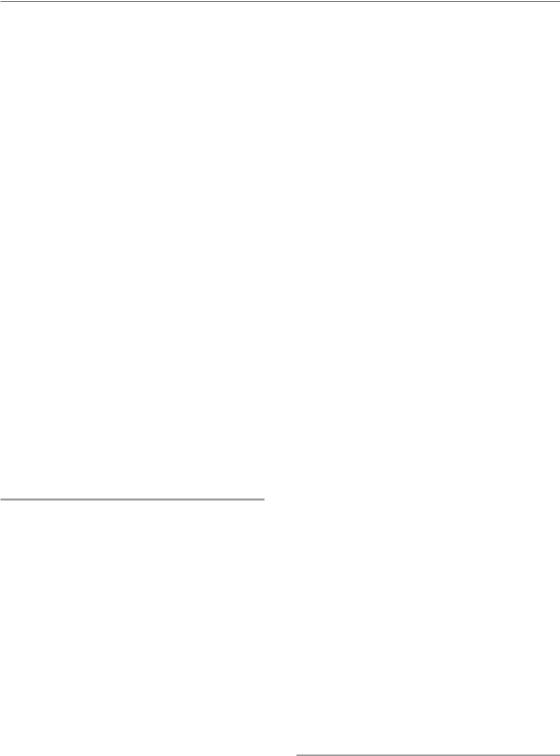
Using PET images for treatment planning 281
PET-avid disease was also excluded from the PTV defined on CT, which would have resulted in a geographical miss. In 16 patients confirming the possible role of FDG-PET/CT in radiotherapy treatment planning for oesophageal cancer (Howard et al., 2004), CT-derived GTVs were compared with GTVs contoured on PET/CT images by means of a conformality index (CI). The mean CI was 0.46, suggesting a significant lack of overlap between the GTVs in a large proportion of patients. The use of PET/CT in treatment planning for patients with oesophageal cancer is now being evaluated in a prospective trial. Another preliminary finding on incorporating EUS and PET scanning in the treatment planning process of 25 patients with oesophageal carcinoma showed that the measured tumour length was significantly longer on CT than on PET with FDG (Konski et al., 2005). The authors concluded that PET could be of additional help in the treatment planning. Although EUS measurements of the tumour length were as accurate as PET measurements, the results of EUS are difficult to translate into the planning process. A major drawback of this study was the lack of comparison with pathology findings after surgery.
Rectal cancer
The usefulness of FDG-PET for the initial staging of colorectal cancer has been investigated. Preoperative PET may be useful for the diagnosis of the primary tumour, but it is of limited value for detecting metastasis to the regional lymph nodes (Table 20.1). The potential use of PET/CT in radiotherapy planning for rectal cancer has been addressed in only one study (Ciernik et al., 2005) which evaluated the accuracy of target volume definition with FDG-PET in 11 patients using an integrated PET/CT system. They found that the PET-defined GTV did not correlate well with the pathologic tumour volume. However, this study should be interpreted with caution, as there are several methodological weaknesses. First, pretreatment PET/CT was compared with pathological specimens obtained after preoperative chemoradiotherapy. Second, a fixed threshold was used and a so-called ‘standardized region-growing
algorithm’ to segment the target volumes. This procedure omits the selection and delineation of the CTV, which contains all pelvic regions that are at risk for subclinical disease in rectal cancer, including the internal iliac lymph node regions (Roels et al., 2006).
Although FDG-PET has a high sensitivity for colorectal cancer, there are some limitations in specificity, mainly owing to FDG uptake by macrophages. This limitation becomes important when assessing tumour volume during treatment or when assessing response to chemoradiation, as radiation induces mucositis of the rectal wall surrounding the tumour. In this regard, efforts are being made to develop new 18F-labelled tracers that might be more tumour specific.
Even if PET can provide additional functional information, its usefulness in the treatment of rectal cancer patients is still questionable and needs to be evaluated in prospective trials using a strict methodology. Its benefit might be of little interest in preoperative 3D-CRT, as the total mesorectum included in the CTV will be surgically removed anyway. However, it may become more important when ‘dose painting’ to relevant biological regions is achieved with simultaneous integrated boost techniques. Whether this in turn can improve patient outcome in terms of local control and/or sphincter preservation has to be tested in future trials. Moreover, there remain problems that require specific attention, such as image co-registration and variations in patient setup, in organ motion (e.g. bowel movements) and in organ shape (e.g. bladder filling). The use of an integrated PET/CT is the modality of choice when it comes to more accurate registration in this site. However, there are still small variations possible because of the elastic properties of the rectal wall resulting in distortions of the rectum (and tumour) during the time of acquisition. Displacements of the rectal wall/tumour could also induce geographical misses when dose escalation to small volumes is planned.
Cervix cancer
As already mentioned in Section 20.1 (Table 20.1), FDG-PET appears to be of particular value in detecting para-aortic lymph node involvement.
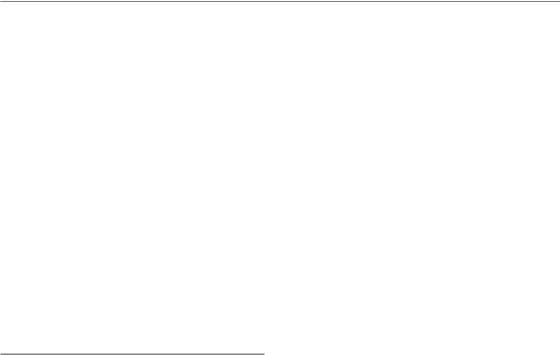
282 Molecular image-guided radiotherapy with positron emission tomography
Based on these findings, a proof-of-concept study has been reported on four patients in whom FDGPET was used to guide intensity-modulated radiation therapy (IMRT) treatments by altering the GTV selection with inclusion of para-aortic lymph nodes (Mutic et al., 2003). FluorodeoxyglucosePET has also been used to delineate the GTV (fixed threshold of 40 per cent of the maximum activity) in 24 patients treated by brachytherapy and external 3D-CRT (Lin et al., 2005). This preliminary study has indicated the benefit of FDG-PET in improving the coverage of large tumours. However, confirmatory studies on larger series of patients are needed before it is possible to ascertain the exact role of FDG-PET for volume delineation of cervix tumours.
20.5 THERAGNOSTIC IMAGING FOR RADIATION ONCOLOGY
Recent developments in molecular imaging have created opportunities to probe the heterogeneity of the tumour biology. In addition to FDG, which is likely to be a surrogate of tumour burden and hence of clonogen density, tracers of hypoxia, such as fluoromisonidazole (F-Miso), 3,3,3-trifluoro- propylamine (EF3), fluoro-erythronitroimidazole (FETNIM) or copper-diacetyl-bisN4-methylth- iosemicarbazone (Cu-ATSM), of proliferation such as 5-bromo-2 -fluoro-2 -deoxyuridine (BFU) or 3 -deoxy-3 -fluorothymidine (FLT), and of receptor expression such as epithelial growth factor receptor (EGFR), have been developed.
Among the PET tracers for hypoxia, F-Miso is the most widely reported but no studies have yet used the tracer to actually define a sub-GTV, which would benefit from a higher radiation dose. There are still several questions that remain open regarding the use of these hypoxia tracers for treatment planning purpose. For example, it is still unclear whether all the tracers are imaging similar biological characteristics, and thus could be interchangeable. In a recent study comparing F-Miso and EF3 in mouse tumour models, it has been shown that these two tracers might not have the same specificity for cellular hypoxia, and thus may not identify identical hypoxic fractions within a GTV (Mahy et al., 2008).
Fluorothymidine (FLT) is a tracer which images DNA synthesis and cellular proliferation by entering the salvage pathway of DNA synthesis. It is thus not incorporated into DNA as the native nucleotides. Amino acids, like FLT, are of interest because they do not seem to accumulate in inflammatory processes and could therefore provide a more specific tumour label (Been et al., 2004). Comparison between FLT and FDG-PET imaging in colorectal cancer has demonstrated lower standardized uptake value (SUV) values for FLT compared with FDG. No correlation was found between the uptake of both tracers, confirming that FLT and FDG detect distinct processes. It has been found that FLT is a valuable tracer for improvement of the specificity for the detection of colorectal tumours. The lack of FLT uptake in inflammatory cells, which are present during and after radiotherapy and/or chemotherapy, makes FLT a promising agent to measure the response to anti-cancer therapy. Preclinical and clinical studies in colorectal cancer show a good correlation between FLT uptake and proliferation (Francis et al., 2004). However, as FLT is not incorporated into DNA, results of clinical PET studies with FLT should be interpreted carefully, taking into account previous treatment and bearing in mind that FLT uptake observed in vivo might result from various mechanisms with possibly divergent influences.
Although limitations certainly exist regarding the use of these various tracers, their integration into the treatment planning process could give a clearer view of the biological pathways involved in radiation response, and hence could be used to ‘paint’ or ‘sculpt’ the dose in various subvolumes using IMRT. Along this line of investigation, ‘dummy run’ studies have been reported with FDG and Cu-ATSM (Chao et al., 2001; De Ruysscher et al., 2005a). Although these studies demonstrate the feasibility of the concept of dose-painting, no clinical validation has been undertaken.
In all the studies on target volume definition to date, it has been assumed that, even when defined with regard to specific biological pathways, GTVs were homogeneous and did not vary during the course of a radiation treatment. Hence, a radiation dose homogeneously distributed in space and time is delivered (i.e. a so-called four-dimensional
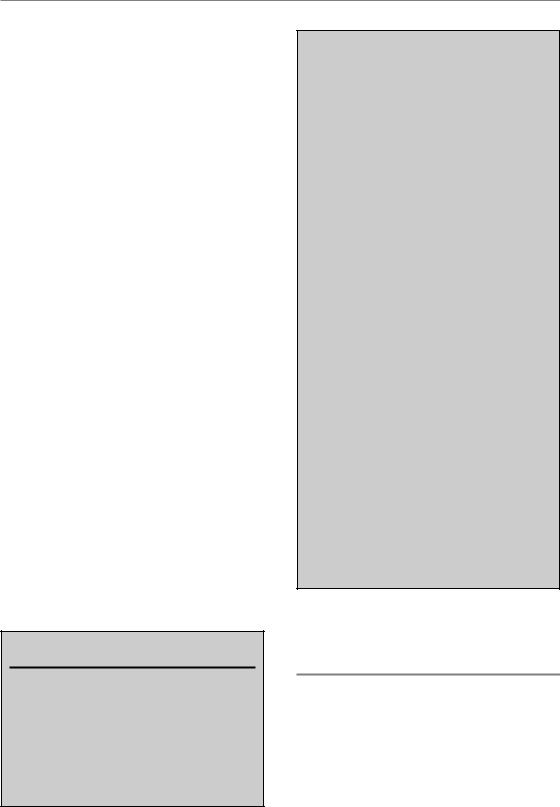
Bibliography 283
homogeneous dose distribution. This is likely to be an oversimplification of the biological reality, as tumours are known to be heterogeneous with respect to pathways of importance for radiation response, and are also known to progressively shrink, at least some of them, during treatment.
Bentzen (2005) has proposed the term ‘theragnostic’ to describe the use of molecular imaging to assist in prescribing the distribution of radiation dose in four dimensions (i.e. the three spatial dimensions plus time). This is undoubtedly a challenging research topic, which could potentially revolutionize the process of radiotherapy planning and delivery. However, several issues need to be resolved. From a planning point of view, the challenge will be to establish the correspondence between a PET signal intensity (or a PET image segmentation) and a prescribed dose, thus evolving the concept of dose-painting into dose-painting by number (Alber et al., 2003). We will also need to develop the tools to register in space and time the various images and the dose distributions acquired throughout the therapy. To this end, non-rigid registration techniques will be required as tumour and/or normal-tissue shrinkage is expected during the course of a radiotherapy treatment. From a biological viewpoint, the challenge will be to relate a change in tracer uptake to a change in the underlying biology, thus requiring a comprehensive biological validation of the concept of dose-painting or dose-painting by number in experimental models. However, although much research still needs to be done, it is likely that during the next 10 years these concepts and procedures will be increasingly incorporated into clinical radiotherapy.
types. Given the considerable range of PET accuracies across different tumour types, its role will not be identical in the different tumour locations.
3.In using PET for volume segmentation, image acquisition, reconstruction and analysis need new standards favouring image quality over reconstruction speed.
4.Observer-independent segmentation of PET images is required for automatic delineation of the GTV. Gradient-based methods appear more robust than threshold-based methods, especially in difficult cases (low uptake and/or peritumoural inflammation) encountered during radiotherapy treatment.
5.The use of molecular imaging for treatment planning is under validation in various disease sites. It has shown encouraging results in head and neck and lung tumours.
6.‘Theragnostic’ has been proposed to describe the use of molecular imaging to assist in prescribing the distribution of radiation dose in four dimensions – the three spatial dimensions plus time. It is a challenging concept at the frontier between radiation oncology, radiation biology and radiation physics that still requires thorough testing.
7.Before proper validation of the use of various PET tracers has been performed, and all methodological aspects have been fully optimized, it is reasonable to say that the use of PET for treatment planning purposes should not be used on a routine basis, but should remain in the clinical research arena.
Key points
1.Molecular imaging is the use of non-inva- sive imaging techniques (e.g. PET, fMRI) that enable the visualization of various biological pathways and physiological characteristics of tumours and normal tissues.
2.FDG-PET may be of benefit for the selection of target volumes depending on its sensitivity and specificity for various tumour
■ BIBLIOGRAPHY
Abdel-Nabi H, Doerr RJ, Lamonica DM et al. (1998). Staging of primary colorectal carcinomas with fluorine-18 fluorodeoxyglucose whole-body PET: correlation with histopathologic and CT findings.
Radiology 206: 755–60.
Alber M, Paulsen F, Eschmann SM, Machulla HJ (2003). On biologically conformal boost dose optimization.
Phys Med Biol 48: N31–5.

284 Molecular image-guided radiotherapy with positron emission tomography
Antoch G, Saoudi N, Kuehl H et al. (2004). Accuracy of whole-body dual-modality fluorine-18–2-fluoro- 2-deoxy-D-glucose positron emission tomography and computed tomography (FDG-PET/CT) for tumor staging in solid tumors: comparison with CT and PET.
J Clin Oncol 22: 4357–68.
Ashamalla H, Rafla S, Parikh K et al. (2005). The contribution of integrated PET/CT to the evolving definition of treatment volumes in radiation treatment planning in lung cancer. Int J Radiat Oncol Biol Phys 63: 1016–23.
Bachaud JM, Marre D, Dygai I et al. (2005). [The impact of 18F-fluorodeoxyglucose positron emission tomography on the 3D conformal radiotherapy planning in patients with non-small cell lung cancer]. Cancer Radiother 9: 602–9.
Bar-Shalom R, Yefremov N, Guralnik L et al. (2003). Clinical performance of PET/CT in evaluation of cancer: additional value for diagnostic imaging and patient management. J Nucl Med 44: 1200–9.
Been LB, Suurmeijer AJ, Cobben DC, Jager PL, Hoekstra HJ, Elsinga PH (2004). [18F]FLT-PET in oncology: current status and opportunities. Eur J Nucl Med Mol Imaging 31: 1659–72.
Bentzen SM (2005). Theragnostic imaging for radiation oncology: dose-painting by numbers. Lancet Oncol 6: 112–7.
Birim O, Kappetein AP, Stijnen T, Bogers AJ (2005). Meta-analysis of positron emission tomographic and computed tomographic imaging in detecting mediastinal lymph node metastases in nonsmall cell lung cancer. Ann Thorac Surg 79: 375–82.
Boucher L, Rodrigue S, Lecomte R, Benard F (2004). Respiratory gating for 3-dimensional PET of the thorax: feasibility and initial results. J Nucl Med 45: 214–9.
Chao KS, Bosch WR, Mutic S et al. (2001). A novel approach to overcome hypoxic tumor resistance: Cu-ATSM-guided intensity-modulated radiation therapy. Int J Radiat Oncol Biol Phys 49: 1171–82.
Ciernik IF, Dizendorf E, Baumert BG et al. (2003). Radiation treatment planning with an integrated positron emission and computer tomography (PET/CT): a feasibility study. Int J Radiat Oncol Biol Phys 57: 853–63.
Ciernik IF, Huser M, Burger C, Davis JB, Szekely G (2005). Automated functional image-guided radiation treatment planning for rectal cancer. Int J Radiat Oncol Biol Phys 62: 893–900.
Daisne JF, Duprez T, Weynand B et al. (2004). Tumor volume in pharyngolaryngeal squamous cell carcinoma: comparison at CT, MR imaging, and FDG PET and validation with surgical specimen.
Radiology 233: 93–100.
De Ruysscher D, Wanders S, Minken A et al. (2005a). Effects of radiotherapy planning with a dedicated combined PET-CT-simulator of patients with nonsmall cell lung cancer on dose limiting normal tissues and radiation dose-escalation: a planning study. Radiother Oncol 77: 5–10.
De Ruysscher D, Wanders S, van Haren E et al. (2005b). Selective mediastinal node irradiation based on FDG-PET scan data in patients with non-small-cell lung cancer: a prospective clinical study. Int J Radiat Oncol Biol Phys 62: 988–94.
Erdi YE, Mawlawi O, Larson SM et al. (1997). Segmentation of lung lesion volume by adaptive positron emission tomography image thresholding. Cancer 80: 2505–9.
Fox JL, Rengan R, O’Meara W et al. (2005). Does registration of PET and planning CT images decrease interobserver and intraobserver variation in delineating tumor volumes for non-small-cell lung cancer? Int J Radiat Oncol Biol Phys 62: 70–5.
Francis DL, Visvikis D, Costa DC et al. (2004). Assessment of recurrent colorectal cancer following 5-fluorouracil chemotherapy using both
18FDG and 18FLT PET. Eur J Nucl Med Mol Imaging
31: 928.
Geets X, Daisne JF, Arcangeli S et al. (2005). Interobserver variability in the delineation of pharyngolaryngeal tumor, parotid glands and cervical spinal cord: comparison between CT-scan and MRI.
Radiother Oncol 77: 25–31.
Geets X, Daisne JF, Tomsej M, Duprez T, Lonneux M, Gregoire V (2006). Impact of the type of imaging modality on target volumes delineation and dose distribution in pharyngo-laryngeal squamous cell carcinoma: comparison between preand pertreatment studies. Radiother Oncol 78: 291–7.
Geets X, Lee JA, Bol A, Lonneux M, Gregoire V (2007a). A gradient-based method for segmenting FDG-PET images: methodology and validation. Eur J Nucl Med Mol Imaging 34: 1427–38.
Geets X, Tomsej M, Lee JA (2007b). Adaptive biological image-guided IMRT with anatomic and functional imaging in pharyngo-laryngeal tumors: impact on target volume delineation and dose distribution

Bibliography 285
using helical tomotherapy. Radiother Oncol 85: 105–15.
Gregoire V, Daisne JF, Geets X (2005). Comparison of CTand FDG-PET-defined GT. Int J Radiat Oncol Biol Phys 63: 308–9.
Grigsby PW, Siegel BA, Dehdashti F (2001). Lymph node staging by positron emission tomography in patients with carcinoma of the cervix. J Clin Oncol 19:
3745–9.
Grosu AL, Lachner R, Wiedenmann N et al. (2003). Validation of a method for automatic image fusion (BrainLAB System) of CT data and 11C-methionine- PET data for stereotactic radiotherapy using a LINAC: first clinical experience. Int J Radiat Oncol Biol Phys 56: 1450–63.
Grosu AL, Piert M, Weber WA et al. (2005). Positron emission tomography for radiation treatment planning. Strahlenther Onkol 181: 483–99.
Herholz K, Holzer T, Bauer B et al. (1998). 11C-methionine PET for differential diagnosis of low-grade gliomas.
Neurology 50: 1316–22.
Howard A, Mehta MP, Ritter MA et al. (2004). The value of PET/CT in gross tumor volume delineation in lung and esophagus cancer. Int J Radiat Oncol Biol Phys
60(Suppl. 1): S536–7.
Jacobs AH, Winkler A, Dittmar C et al. (2002). Molecular and functional imaging technology for the development of efficient treatment strategies for gliomas. Technol Cancer Res Treat 1: 187–204.
Konski A, Doss M, Milestone B, Haluszka O, Hanlon A, Freedman G, Adler L (2005). The integration of 18- fluoro-deoxy-glucose positron emission tomography and endoscopic ultrasound in the treatmentplanning process for esophageal carcinoma. Int J Radiat Oncol Biol Phys 61: 1123–8.
Kracht LW, Miletic H, Busch S et al. (2004). Delineation of brain tumor extent with [11C]L-methionine positron emission tomography: local comparison with stereotactic histopathology. Clin Cancer Res 10: 7163–70.
Leong T, Everitt C, Yuen K et al. (2004). A prospective study to evaluate the impact of coregistered PET/CT images on radiotherapy treatment planning for esophageal cancer. Int J Radiat Oncol Biol Phys
60(Suppl. 1): S139–40.
Lin LL, Mutic S, Malyapa RS et al. (2005). Sequential FDG-PET brachytherapy treatment planning in carcinoma of the cervix. Int J Radiat Oncol Biol Phys
63: 1494–501.
Mahy P, de Bast M, de Groot T et al. (2008). Comparative pharmacokinetics, biodistribution, metabolism and hypoxia-dependent uptake of [18F]-EF3 and [18F]-MISO in rodent tumors model.
Radiother Oncol (in press).
Menda Y, Graham MM (2005). Update on 18F- fluorodeoxyglucose/positron emission tomography and positron emission tomography/computed tomography imaging of squamous head and neck cancers. Semin Nucl Med 35: 214–9.
Moureau-Zabotto L, Touboul E, Lerouge D et al. (2005). Impact of CT and 18F-deoxyglucose positron emission tomography image fusion for conformal radiotherapy in esophageal carcinoma. Int J Radiat Oncol Biol Phys 63: 340–5.
Mutic S, Malyapa RS, Grigsby PW et al. (2003). PETguided IMRT for cervical carcinoma with positive para-aortic lymph nodes: a dose-escalation treatment planning study. Int J Radiat Oncol Biol Phys 55: 28–35.
Nagayoshi M, Murase K, Fujino K et al. (2005). Usefulness of noise adaptive non-linear Gaussian filter in FDG-PET study. Ann Nucl Med 19: 469–77.
Nestle U, Kremp S, Schaefer-Schuler A et al. (2005). Comparison of different methods for delineation of 18F-FDG PET-positive tissue for target volume definition in radiotherapy of patients with nonsmall cell lung cancer. J Nucl Med 46: 1342–8.
Nuutinen J, Sonninen P, Lehikoinen P et al. (2000). Radiotherapy treatment planning and long-term follow-up with [(11)C]methionine PET in patients with low-grade astrocytoma. Int J Radiat Oncol Biol Phys 48: 43–52.
Paulino AC, Koshy M, Howell R, Schuster D, Davis LW (2005). Comparison of CTand FDG-PET-defined gross tumor volume in intensity-modulated radiotherapy for head-and-neck cancer. Int J Radiat Oncol Biol Phys 61: 1385–92.
Reinhardt MJ, Ehritt-Braun C, Vogelgesang D et al. (2001). Metastatic lymph nodes in patients with cervical cancer: detection with MR imaging and FDG PET. Radiology 218: 776–82.
Roels S, Duthoy W, Haustermans K et al. (2006). Definition and delineation of the clinical target volume for rectal cancer. Int J Radiat Oncol Biol Phys
65: 1129–42.
Rose PG, Adler LP, Rodriguez M, Faulhaber PF, AbdulKarim FW, Miraldi F (1999). Positron emission tomography for evaluating para-aortic nodal
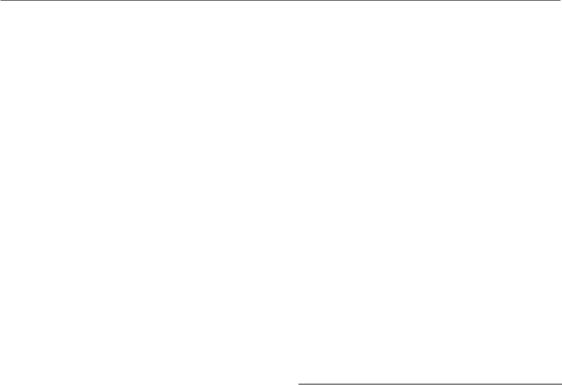
286 Molecular image-guided radiotherapy with positron emission tomography
metastasis in locally advanced cervical cancer before surgical staging: a surgicopathologic study. J Clin Oncol 17: 41–5.
Schmidt D, Gottwald U, Langen KJ et al. (2001). 3-[123I]Iodo-alpha-methyl-L-tyrosine uptake in cerebral gliomas: relationship to histological grading and prognosis. Eur J Nucl Med 28: 855–61.
Schwartz DL, Ford EC, Rajendran J et al. (2005). FDG- PET/CT-guided intensity modulated head and neck radiotherapy: a pilot investigation. Head Neck 27: 478–87.
Shah JP (1990). Patterns of cervical lymph node metastasis from squamous carcinomas of the upper aerodigestive tract. Am J Surg 160: 405–9.
Stuckensen T, Kovacs AF, Adams S, Baum RP (2000). Staging of the neck in patients with oral cavity squamous cell carcinomas: a prospective comparison of PET, ultrasound, CT and MRI. J Craniomaxillofac Surg 28: 319–24.
Sugawara Y, Eisbruch A, Kosuda S, Recker BE, Kison PV, Wahl RL (1999). Evaluation of FDG PET in patients with cervical cancer. J Nucl Med 40: 1125–31.
Sweeney RA, Bale RJ, Moncayo R et al. (2003). Multimodality cranial image fusion using external markers applied via a vacuum mouthpiece and a case report. Strahlenther Onkol 179: 254–60.
Tarantola G, Zito F, Gerundini P (2003). PET instrumentation and reconstruction algorithms in whole-body applications. J Nucl Med 44: 756–69.
Vanderstraeten B, Duthoy W, De Gersem W, De Neve W, Thierens H (2006). [18F]fluoro-deoxy-glucose positron emission tomography ([18F]FDG-PET) voxel intensity-based intensity-modulated radiation therapy (IMRT) for head and neck cancer. Radiother Oncol 79: 249–58.
van der Wel A, Nijsten S, Hochstenbag M et al. (2005). Increased therapeutic ratio by 18FDG-PET CT planning in patients with clinical CT stage N2-N3M0 non-small-cell lung cancer: a modeling study. Int J Radiat Oncol Biol Phys 61: 649–55.
van Westreenen HL, Westerterp M, Bossuyt PM et al. (2004). Systematic review of the staging performance of 18F-fluorodeoxyglucose positron emission tomography in esophageal cancer. J Clin Oncol 22: 3805–12.
Vrieze O, Haustermans K, De Wever W et al. (2004). Is there a role for FGD-PET in radiotherapy planning in esophageal carcinoma? Radiother Oncol 73: 269–75.
Weber WA, Wester HJ, Grosu AL et al. (2000). O-(2-[18F]fluoroethyl)-L-tyrosine and L-[methyl- 11C]methionine uptake in brain tumours: initial results of a comparative study. Eur J Nucl Med 27: 542–9.
Yaremko B, Riauka T, Robinson D, Murray B, McEwan A, Roa W (2005). Threshold modification for tumour imaging in non-small-cell lung cancer using positron emission tomography. Nucl Med Commun 26: 433–40.
■ FURTHER READING
Apisarnthanarax S, Chao KS (2005). Current imaging paradigms in radiation oncology. Radiat Res 163: 1–25.
Bradbury M, Hricak H (2005). Molecular MR imaging in oncology. Magn Reson Imaging Clin N Am 13: 225–40.
Chapman JD, Schneider RF, Urbain JL, Hanks GE (2001). Single-photon emission computed tomography and positron-emission tomography assays for tissue oxygenation. Semin Radiat Oncol 11: 47–57.
Rohren EM, Turkington TG, Coleman RE (2004). Clinical applications of PET in oncology. Radiology 231: 305–32.
van de Wiele C, Lahorte C, Oyen W et al. (2003). Nuclear medicine imaging to predict response to radiotherapy: a review. Int J Radiat Oncol Biol Phys
55: 5–15.