
Molecular Fluorescence
.pdf
2.2 Probability of transitions. The Beer–Lambert Law. Oscillator strength 23
e ect). Butadiene and benzene are the simplest cases of linear and cyclic conjugated systems, respectively.
Because there is no overlap between the s and p orbitals, the p electron system can be considered as independent of the s bonds. It is worth remembering that the greater the extent of the p electron system, the lower the energy of the low-lying p ! p transition, and consequently, the larger the wavelength of the corresponding absorption band. This rule applies to linear conjugated systems (polyenes) and cyclic conjugated systems (aromatic molecules).
2.2
Probability of transitions. The Beer–Lambert Law. Oscillator strength
Experimentally, the e ciency of light absorption at a wavelength l by an absorbing medium is characterized by the absorbance AðlÞ or the transmittance TðlÞ, defined as
I0
AðlÞ ¼ log l ¼ log TðlÞ
Il
TðlÞ ¼ Il
ð2:1Þ
Il0
where Il0 and Il are the light intensities of the beams entering and leaving the absorbing medium, respectively2).
In many cases, the absorbance of a sample follows the Beer–Lambert Law
I0
AðlÞ ¼ log l ¼ eðlÞlc ð2:2Þ
Il
where eðlÞ is the molar (decadic) absorption coe cient (commonly expressed in L mol 1 cm 1), c is the concentration (in mol L 1) of absorbing species and l is the absorption path length (thickness of the absorbing medium) (in cm). Derivation of the Beer–Lambert Law is given in Box 2.1.
2)The term intensity is commonly used but is imprecise. According to IUPAC recommendations (see Pure & Appl. Chem. 68, 2223– 2286 (1996)), this term should be replaced by the spectral radiant power Pl, i.e. the radiant power at wavelength l per unit wavelength
interval. Radiant power is synonymous with radiant (energy) flux. The SI unit for radiant power is J s 1 ¼ W; the SI unit for spectral radiant power is W m 1, but a commonly used unit is W nm 1.
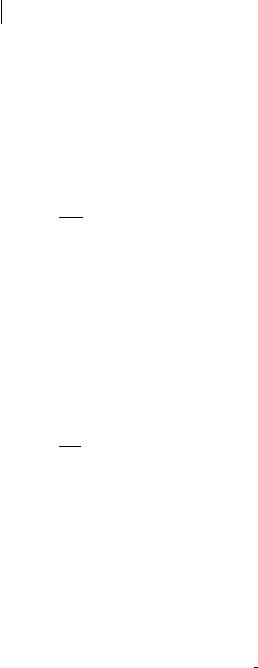
24 2 Absorption of UV–visible light
Failure to obey the linear dependence of the absorbance on concentration, according to the Beer–Lambert Law, may be due to aggregate formation at high concentrations or to the presence of other absorbing species.
Various terms for characterizing light absorption can be found in the literature. The recommendations of the International Union of Pure and Applied Chemistry (IUPAC)3) are very helpful here. In particular, the term optical density, synonymous with absorbance, is not recommended. Also, the term molar absorption coe cient should be used instead of molar extinction coe cient.
The (decadic) absorption coe cient aðlÞ is the absorbance divided by the optical path length, l:
a l |
AðlÞ |
1 |
log |
Il0 |
or I |
|
I0 |
10 aðlÞl |
|||
|
|
|
|
|
|||||||
l |
¼ l |
Il |
l ¼ |
||||||||
ð Þ ¼ |
|
|
l |
|
Physicists usually prefer to use the Napierian absorption coe cient aðlÞ
a l |
1 |
ln |
Il0 |
a l |
Þ |
ln 10 or I |
l ¼ |
I0e aðlÞl |
|
|
|
Il |
|||||||
ð Þ ¼ l |
|
¼ ð |
|
l |
ð2:3Þ
ð2:4Þ
Because absorbance is a dimensionless quantity, the SI unit for a and a is m 1, but cm 1 is often used.
Finally, the molecular absorption cross-section sðlÞ characterizes the photon-cap- ture area of a molecule. Operationally, it can be calculated as the (Napierian) absorption coe cient divided by the number N of molecular entities contained in a
unit volume of the absorbing medium along the light path: |
|
|
||||
s |
l |
Þ ¼ |
aðlÞ |
ð |
2:5 |
|
N |
||||||
ð |
|
Þ |
The relationship between the molecular absorption cross-section and the molar absorption coe cient is described in Box 2.1.
The molar absorption coe cient, eðlÞ, expresses the ability of a molecule to absorb light in a given solvent. In the classical theory, molecular absorption of light can be described by considering the molecule as an oscillating dipole, which allows us to introduce a quantity called the oscillator strength, which is directly related to the integral of the absorption band as follows:
f |
2303 |
mc02 |
ð e n dn |
4:32 10 |
9 |
ð e n dn |
2:6 |
||||||||
|
|
2 |
|
|
|
|
|
|
|
|
|
|
|
|
|
¼ |
|
|
ð |
|
Þ |
|
¼ |
|
|
ð |
|
Þ |
|
|
ð Þ |
|
Nape n |
n |
|
where m and e are the mass and the charge of an electron, respectively, c0 is the speed of light, n is the index of refraction, and n is the wavenumber (in cm 1). f is a dimensionless quantity and values of f are normalized so that its maximum
3)See the Glossary of Terms Used in Photochemistry published in Pure & Appl. Chem. 68, 2223–2286 (1996).
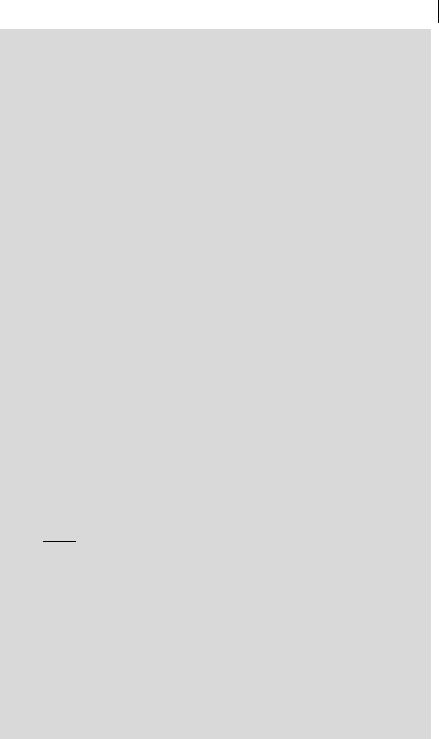
2.2 Probability of transitions. The Beer–Lambert Law. Oscillator strength 25
Box 2.1 Derivation of the Beer–Lambert Law and comments on its practical use
Derivation of the Beer–Lambert Law from considerations at a molecular scale is more interesting than the classical derivation (stating that the fraction of light absorbed by a thin layer of the solution is proportional to the number of absorbing molecules). Each molecule has an associated photon-capture area, called the molecular absorption cross-section s, that depends on the wavelength. A thin layer of thickness dl contains dN molecules. dN is given by
dN ¼ NacS dl
where S is the cross-section of the incident beam, c is the concentration of the solution and Na is Avogadro’s number. The total absorption cross-section of the thin layer is the sum of all molecular cross-sections, i.e. s dN. The probability of photon capture is thus s dN=S and is simply equal to the fraction of light ð dI=IÞ absorbed by the thin layer:
|
dI |
¼ |
s dN |
¼ Nasc dl |
|
I |
|
S |
Integration leads to
ln |
I0 |
¼ Nascl |
or log |
I0 |
¼ |
1 |
Nascl |
I |
I |
2:303 |
where l is the thickness of the solution. This equation is formally identical to Eq. (2.2) with e ¼ Nas=2:303.
The molecular absorption cross-section can then be calculated from the experimental value of e using the following relation:
s ¼ 2:303e ¼ 3:825 10 19e ðin cm2Þ
Na
Practical use of the Beer–Lambert law deserves attention. In general, the sample is a cuvette containing a solution. The absorbance must be characteristic of the absorbing species only. Therefore, it is important to note that in the Beer–Lam- bert Law ðAðlÞ ¼ log I0=I ¼ eðlÞlcÞ, I0 is the intensity of the beam entering the solution but not that of the incident beam Ii on the cuvette, and I is the intensity of the beam leaving the solution but not that of the beam IS leaving the cuvette (see Figure B2.1). In fact, there are some reflections on the cuvette walls and these walls may also absorb light slightly. Moreover, the solvent is assumed to have no contribution, but it may also be partially responsible for a decrease in intensity because of scattering and possible absorption. The contributions of the
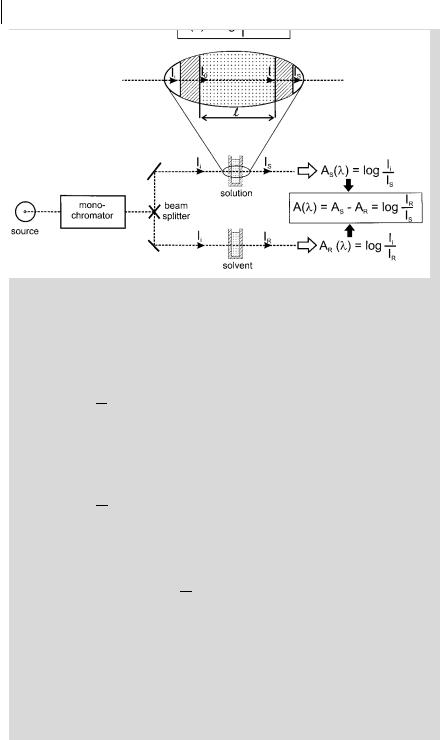
26 2 Absorption of UV–visible light
Fig. B2.1. Practical aspects of absorbance measurements.
cuvette walls and the solvent can be taken into account in the following way. The absorbance of the whole sample (including the cuvette walls) is defined as
ASðlÞ ¼ log Ii
IS
If the solution is replaced by the solvent, the intensity of the transmitted light is IR and the absorbance becomes
ARðlÞ ¼ log Ii
IR
The true absorbance of the solution is then given by
AðlÞ ¼ ASðlÞ ARðlÞ ¼ log IR
IS
As shown in Figure B2.1, double-beam spectrophotometers automatically record the true absorbance by measuring logðIR=ISÞ, thanks to a double compartment containing two cuvettes, one filled with the solution and one filled with the solvent. Because the two cuvettes are never perfectly identical, the baseline of the instrument is first recorded (with both cuvettes filled with the solvent) and stored. Then, the solvent of the sample cuvette is replaced by the solution, and the true absorption spectrum is recorded.

2.2 Probability of transitions. The Beer–Lambert Law. Oscillator strength 27
Tab. 2.1. Examples of molar absorption coefficients, e (at the wavelength corresponding to the maximum of the absorption band of lower energy). Only approximate values are given, because the value of e slightly depends on the solvent
Compound |
e/L mol 1 cm 1 |
Compound |
e/L mol 1 cm 1 |
Benzene |
A200 |
Acridine |
A12 000 |
Phenol |
A2 000 |
Biphenyl |
A16 000 |
Carbazole |
A4 200 |
Bianthryl |
A24 000 |
1-Naphthol |
A5 400 |
Acridine orange |
A30 000 |
Indole |
A5 500 |
Perylene |
A34 000 |
Fluorene |
A9 000 |
Eosin Y |
A90 000 |
Anthracene |
A10 000 |
Rhodamine B |
A105 000 |
Quinine sulfate |
A10 000 |
|
|
|
|
|
|
value is 1. For n ! p transitions, the values of e are in the order of a few hundreds or less and those of f are no greater than @10 3. For p ! p transitions, the values of e and f are in principle much higher (except for symmetry-forbidden transitions): f is close to 1 for some compounds, which corresponds to values of e that are of the order of 105. Table 2.1 gives some examples of values of e.
In the quantum mechanical approach, a transition moment is introduced for characterizing the transition between an initial state and a final state (see Box 2.2). The transition moment represents the transient dipole resulting from the displacement of charges during the transition; therefore, it is not strictly a dipole moment.
The concept of transition moment is of major importance for all experiments carried out with polarized light (in particular for fluorescence polarization experiments, see Chapter 5). In most cases, the transition moment can be drawn as a vector in the coordinate system defined by the location of the nuclei of the atoms4); therefore, the molecules whose absorption transition moments are parallel to the electric vector of a linearly polarized incident light are preferentially excited. The probability of excitation is proportional to the square of the scalar product of the transition moment and the electric vector. This probability is thus maximum when the two vectors are parallel and zero when they are perpendicular.
For p ! p transitions of aromatic hydrocarbons, the absorption transition moments are in the plane of the molecule. The direction with respect to the molecular axis depends on the electronic state attained on excitation. For example, in naphthalene and anthracene, the transition moment is oriented along the short axis for the S0 ! S1 transition and along the long axis for the S0 ! S2 transition. Various examples are shown in Figure 2.3.
4)Note that this is not true for molecules having a particular symmetry, such as benzene ðD6hÞ, triphenylene ðD3hÞ and C60
ðIhÞ.
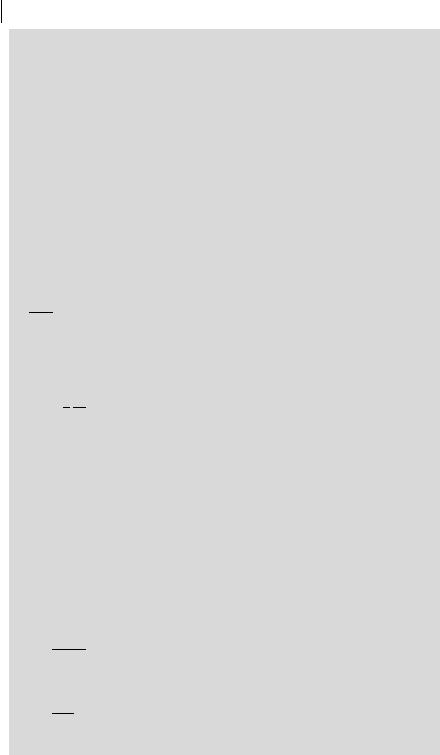
28 2 Absorption of UV–visible light
Box 2.2 Einstein coe cients. Transition moment. Oscillator strength
Let us consider a molecule and two of its energy levels E1 and E2. The Einstein coe cients are defined as follows (Scheme B2.2): B12 is the induced absorption coe cient, B21 is the induced emission coe cient and A21 is the spontaneous emission coe cient.
Scheme B2.2
The coe cients for spontaneous and induced emissions will be discussed in Chapter 3 (see Box 3.2).
The rate at which energy is taken up from the incident light is given by
dPdt12 ¼ B12rðnÞ
where rðnÞ is the energy density incident on the sample at frequency n. B12 appears as the transition rate per unit energy density of the radiation. In the quantum mechanical theory, it is shown that
|
2 p |
B12 ¼ |
3 p2 jhC1jMjC2ij2 ðp ¼ h=2pÞ |
Ð
In this expression, hC1jMjC2i ¼ C1MC2 dt, where C1 and C2 are the wavefunctions of states 1 and 2, respectively, dt is over the whole configuration space of the 3N coordinates, M is the dipole moment operator (M ¼ Serj, where rj is the vector joining the electron j to the origin of a coordinate system linked to the molecule).
It should be noted that the dipole moment of the molecule in its ground state is hC1jMjC1i. In contrast, the term hC1jMjC2i is not strictly a dipole moment because there is a displacement of charges during the transition; it is called transition moment M12 that characterizes the transition.
It is interesting that there is a relation between the oscillator strength f (given by Eq. 2.6) and the square of the transition moment integral, which bridges the gap between the classical and quantum mechanical approaches:
f ¼ 8p2mn jhC1jMjC2ij2
3he2
Note also that the oscillator strength can be related to the coe cient B12:
mhn
f ¼ pe2 B12
For more details, see Birks (1970), pp. 48–52.
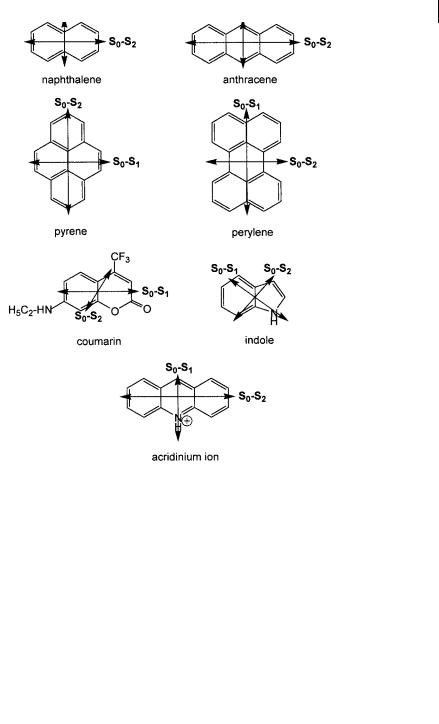
2.2 Probability of transitions. The Beer–Lambert Law. Oscillator strength 29
Fig. 2.3. Examples of molecules with their absorption transition moments.
Scheme 2.1

302 Absorption of UV–visible light
2.3
Selection rules
There are two major selection rules for absorption transitions:
1.Spin-forbidden transitions. Transitions between states of di erent multiplicities are forbidden, i.e. singlet–singlet and triplet–triplet transitions are allowed, but singlet–triplet and triplet–singlet transitions are forbidden. However, there is always a weak interaction between the wavefunctions of di erent multiplicities
via spin–orbit coupling5). As a result, a wavefunction for a singlet (or triplet) state always contains a small fraction of a triplet (or singlet) wavefunction C ¼ a1C þ b3C; this leads to a small but non-negligible value of the intensity integral during a transition between a singlet state and a triplet state or vice versa (see Scheme 2.1). In spite of their very small molar absorption coe cients, such transitions can be e ectively observed.
Intersystem crossing (i.e. crossing from the first singlet excited state S1 to the first triplet state T1) is possible thanks to spin–orbit coupling. The e ciency of this coupling varies with the fourth power of the atomic number, which explains why intersystem crossing is favored by the presence of a heavy atom. Fluorescence quenching by internal heavy atom e ect (see Chapter 3) or external heavy atom e ect (see Chapter 4) can be explained in this way.
2.Symmetry-forbidden transitions. A transition can be forbidden for symmetry reasons. Detailed considerations of symmetry using group theory, and its consequences on transition probabilities, are beyond the scope of this book. It is important to note that a symmetry-forbidden transition can nevertheless be observed because the molecular vibrations cause some departure from perfect symmetry (vibronic coupling). The molar absorption coe cients of these transitions are very small and the corresponding absorption bands exhibit welldefined vibronic bands. This is the case with most n ! p transitions in solvents that cannot form hydrogen bonds (e A100–1000 L mol 1 cm 1).
2.4
The Franck–Condon principle
According to the Born–Oppenheimer approximation, the motions of electrons are much more rapid than those of the nuclei (i.e. the molecular vibrations). Promotion of an electron to an antibonding molecular orbital upon excitation takes about 10 15 s, which is very quick compared to the characteristic time for molecular vi-
5) Spin–orbit coupling can be understood in a |
an axis of its own, which generates another |
primitive way by considering the motion of |
magnetic moment. Spin–orbit coupling |
an electron in a Bohr-like orbit. The rotation |
results from the interaction between these |
around the nucleus generates a magnetic |
two magnets. |
moment; moreover, the electron spins about |
|
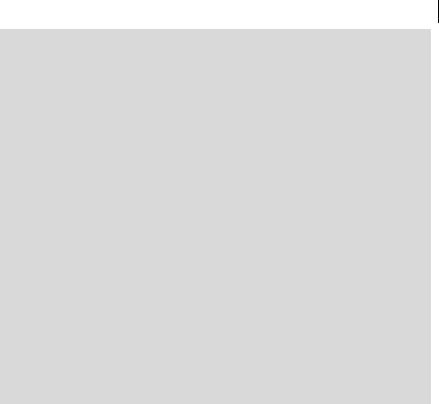
2.4 The Franck–Condon principle 31
Box 2.3 Classical and quantum mechanical description of the Franck–Condon principlea)
Classically, the transition occurs when the distances between nuclei are equal to the equilibrium bond lengths of the molecule in the ground state. While the transition is in progress, the position of the nuclei is unchanged and they do not accelerate. Consequently, the transition terminates where the vertical line intersects the potential energy curve of the lowest excited state, i.e. at the turning point. As soon as the transition is complete, the excited molecule begins to vibrate at an energy corresponding to the intersection.
In the quantum mechanical description (in continuation of Box 2.2), the wavefunction can be described by the product of an electronic wavefunction C and a vibrational wavefunction w (the rotational contribution can be neglected), so that the probability of transition between an initial state defined by C1wa and a final state defined by C2wb is proportional to jhC1wajMjC2wbij2. Because M only depends on the electron coordinates, this expression can be rewritten as the product of two terms jhC1jMjC2ij2jhwa j wbij2 where the second term is called the Franck–Condon factor. Qualitatively, the transition occurs from the lowest vibrational state of the ground state to the vibrational state of the excited state that it most resembles in terms of vibrational wavefunction.
a)Atkins P. W. and Friedman R. S. (1997)
Molecular Quantum Mechanics, Oxford University Press, Oxford.
brations (10 10 –10 12 s). This observation is the basis of the Franck–Condon principle: an electronic transition is most likely to occur without changes in the positions of the nuclei in the molecular entity and its environment (Box 2.3). The resulting state is called a Franck–Condon state, and the transition is called vertical transition, as illustrated by the energy diagram of Figure 2.4 in which the potential energy curve as a function of the nuclear configuration (internuclear distance in the case of a diatomic molecule) is represented by a Morse function.
At room temperature, most of the molecules are in the lowest vibrational level of the ground state (according to the Boltzmann distribution; see Chapter 3, Box 3.1). In addition to the ‘pure’ electronic transition called the 0–0 transition, there are several vibronic transitions whose intensities depend on the relative position and shape of the potential energy curves (Figure 2.4).
The width of a band in the absorption spectrum of a chromophore located in a particular microenvironment is a result of two e ects: homogeneous and inhomogeneous broadening. Homogeneous broadening is due to the existence of a continuous set of vibrational sublevels in each electronic state. Inhomogeneous broadening results from the fluctuations of the structure of the solvation shell

32 2 Absorption of UV–visible light
Fig. 2.4. Top: Potential energy diagrams with vertical transitions (Franck–Condon principle). Bottom: shape of the absorption bands; the vertical broken lines represent the absorption lines that are observed for a vapor, whereas broadening of the spectra is expected in solution (solid line).
surrounding the chromophore. Such broadening e ects exist also for emission bands in fluorescence spectra and will be discussed in Section 3.5.1.
Shifts in absorption spectra due to the e ect of substitution or a change in environment (e.g. solvent) will be discussed in Chapter 3, together with the e ects on emission spectra. Note that a shift to longer wavelengths is called a bathochromic shift (informally referred to as a red-shift). A shift to shorter wavelengths is called a hypsochromic shift (informally referred to as a blue-shift). An increase in the molar absorption coe cient is called the hyperchromic e ect, whereas the opposite is the hypochromic e ect.