
Molecular Fluorescence
.pdf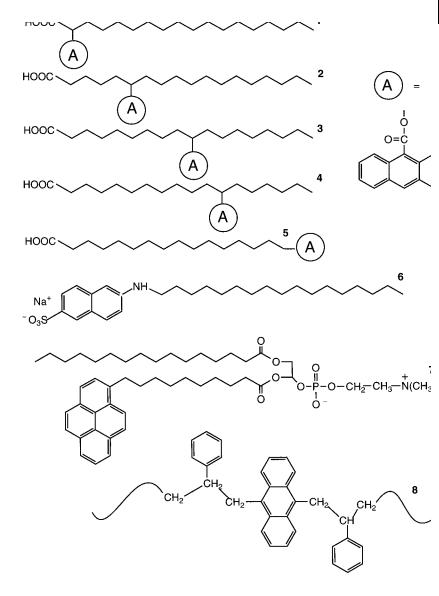
1.4 Fluorescent probes 13
Fig. 1.4. Examples of surfactants, phospholipids and polymers with covalently bound probes. 1: 2-(9-anthroyloxy)stearic acid.
2:6-(9-anthroyloxy)stearic acid.
3:10-(9-anthroyloxy)stearic acid.
4:12-(9-anthroyloxy)stearic acid.
5:(9-anthroyloxy)palmitic acid.
6:2-(N-octadecyl)amino-naphthalene-6-sulfonic acid, sodium salt. 7: 3-palmitoyl-2-(1- pyrenedecanoyl)-L-a-phosphatidylcholine.
8:polystyrene labeled with anthracene.
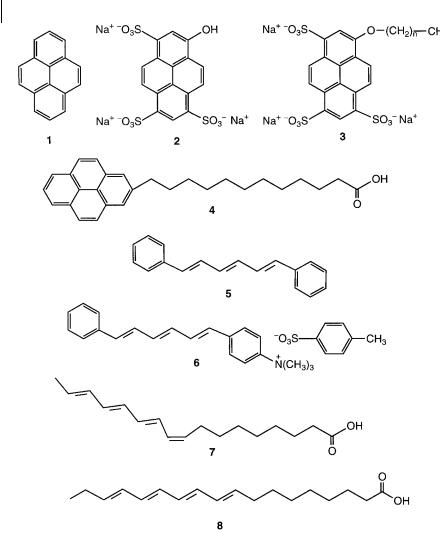
14 1 Introduction
Fig. 1.5. Examples of hydrophobic, hydrophilic and amphiphilic probes. 1: pyrene. 2: 8- hydroxypyrene-1,3,6-trisulfonic acid, trisodium salt (pyranine). 3: 8-alkoxypyrene-1,3,6- trisulfonic acid, trisodium salt. 4: 1-
pyrenedodecanoic acid. 5: 1,6-diphenyl-1,2,5- hexatriene (DPH). 6: 1-(4-trimethylammonium- phenyl)-6-phenyl-1,3,5-hexatriene, p-toluene sulfonate (TMA–DPH). 7: cis-parinaric acid.
8: trans-parinaric acid.
covalently associating probes (class III). The sites of solubilization of extrinsic probes are governed by their chemical nature and the resulting specific interactions that can be established within the region of the system to be probed. The hydrophilic, hydrophobic or amphiphilic character of a probe is essential. Figure 1.5 gives various examples. Pyrene is known as a probe of hydrophobic regions; furthermore its sensitivity to polarity is very useful (see Chapter 7). In contrast, pyranine is very hydrophilic and will be located in hydrophilic aqueous regions;

1.5 Molecular fluorescence as an analytical tool 15
moreover it is sensitive to pH. If the OH group is replaced by Oa(CH2)n aCH3, the resulting molecule becomes pH-insensitive and amphiphilic; the fluorophore moiety plays the role of a polar head and thus is located at the surfactant–water interface of systems consisting of amphiphilic molecules (bilayers of membranes and vesicles, micellar systems, etc.). Conversely, the pyrene moiety of pyrenedodecanoic acid is deeply embedded in the hydrophobic part of an organized assembly. 1,6-Diphenyl-1,3,5-hexatriene (DPH) is located in the hydrocarbon region of bilayers of membrane and vesicles, whereas its cationic analog TMA–DPH is anchored with its charged group at the surfactant–water interface. The latter is thus a probe of the upper region of bilayers. Cisand trans-parinaric acids are good examples of probes causing minimum spatial perturbation to organized assemblies.
The above examples show that a very important criterion in the choice of a probe is its sensitivity to a particular property of the microenvironment in which it is located (e.g. polarity, acidity, etc.). On the other hand, insensitivity to the chemical nature of the environment is preferable in some cases (e.g. in fluorescence polarization or energy transfer experiments). Environment-insensitive probes are also better suited to fluorescence microscopy and flow cytometry.
A criticism often aimed at the use of extrinsic fluorescent probes is the possible local perturbation induced by the probe itself on the microenvironment to be probed. There are indeed several cases of systems perturbed by fluorescent probes. However, it should be emphasized that many examples of results consistent with those obtained by other techniques can be found in the literature (transition temperature in lipid bilayer, flexibility of polymer chains, etc.). To minimize the perturbation, attention must be paid to the size and shape of the probe with respect to the probed region.
In conclusion, the choice of a fluorescent probe is crucial for obtaining unambiguous interpretations. The major aspects that should be taken into consideration are shown in Figure 1.6.
1.5
Molecular fluorescence as an analytical tool
Analytical techniques based on fluorescence detection are very popular because of their high sensitivity and selectivity, together with the advantages of spatial and temporal resolution, and the possibility of remote sensing using optical fibers.
When an analyte is fluorescent, direct fluorometric detection is possible by means of a spectrofluorometer operating at appropriate excitation and observation wavelengths. This is the case for aromatic hydrocarbons (e.g. in crude oils), proteins (e.g. in blood serum, in cow milk), some drugs (e.g. morphine), chlorophylls, etc. Numerous fields of applications have been reported: analysis of air and water pollutants, oils, foods, drugs; monitoring of industrial processes; monitoring of species of clinical relevance; criminology; etc.
However, most ions and molecules are not fluorescent and the main indirect methods that are used in this case are the following:
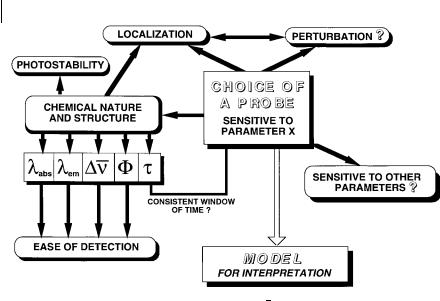
16 1 Introduction
Fig. 1.6. Strategy for the choice of a fluorescent probe. Dn, F, and t are the Stokes shift, quantum yield and lifetime, respectively (see definitions in Chapter 3).
.Derivatization, i.e. reaction of the analyte with a reagent leading to a fluorescent compound, is often used in conjunction with liquid chromatography with fluorescence detection. This method is currently used in biochemistry and clinical
. chemistry.Formation of a fluorescent complex is the basis of most methods of ion and mole- cule recognition (see Chapter 9).
. Fluorescence quenching resulting from the collision of the analyte with a fluorescent compound (see Chapter 4). This method is particularly well suited to the detection of gases such as oxygen (dissolved in water or blood), SO2, H2S, ammonia, HCl, Cl2, chlorocarbons, etc.
Finally, fluorescence immunoassay is a method of major importance for biochemical and biomedical applications.
1.6
Ultimate temporal and spatial resolution: femtoseconds, femtoliters, femtomoles and single-molecule detection
The ability of fluorescence to provide temporal information is of major importance. Great progress has been made since the first determination of an excited-state lifetime by Gaviola in 1926 using a phase fluorometer. A time resolution of a few tens of picosecond can easily be achieved in both pulse and phase fluorometries by using high repetition rate picosecond lasers and microchannel plate photo-

1.6 Ultimate temporal and spatial resolution 17
multipliers (see Chapter 6). Such a time resolution is limited by the response of the photomultiplier but not by the width of the laser pulse, which can be as short as 50–100 fs (1 femtosecond ¼ 10 15 second) (e.g. with a titanium:sapphire laser). The time resolution can be reduced to a few picoseconds with a streak camera. To get an even better time resolution (100–200 fs), a more recent technique based on fluorescence up-conversion has been developed (see Chapter 11).
Regarding spatial resolution, fluorescence microscopy in confocal configuration or with two-photon excitation (see Chapter 11) allows the di raction limit to be approached, which is approximately half the wavelength of the excitation light (0.2–0.3 mm for visible radiation) with the advantage of three-dimensional resolution. The excitation volume can be as small as 0.1 fL (femtoliter). Compared to conventional fluorometers, this represents a reduction by a factor of 1010 of the excitation volume. At high dilution (A10 9 M or less), fluorophores entering and leaving such a small volume cause changes in fluorescence intensity. Analysis of these fluctuations (which is the object of fluorescence correlation spectroscopy; see Chapter 11) in terms of autocorrelation function can provide information on translational di usion, flow rates and molecular aggregation. Fluctuations can also be caused by chemical reactions or rotational di usion. The typical lower limit concentration is @1 fM (femtomol L 1). The progress of these techniques allows us to study molecular interactions at the unsurpassed sensitivity of single-molecule detection.
The di raction limit can be overcome by using a sub-wavelength light source and by placing the sample very close to this source (i.e. in the near field). The relevant domain is near-field optics (as opposed to far-field conventional optics), which has been applied in particular to fluorescence microscopy. This technique, called near-field scanning optical microscopy (NSOM), is an outstanding tool in physical, chemical and life sciences for probing the structure of matter or living systems. The resolution is higher than in confocal microscopy, with the additional capability of force mapping of the surface topography, and the advantage of reduced photobleaching. Single molecule detection is of course possible by this technique.
The first optical detection of a single molecule was reported in 1989 by Moerner and Kador, who detected a single pentacene molecule doped into a p-terphenyl crystal (at liquid helium temperature) using absorption with a double modulation technique. Fluorescence excitation spectroscopy on a single molecule was demonstrated for the first time by Orrit and Bernard in 1990. The detection of a single fluorescent molecule in solution was achieved not much later. Therefore, Schro¨- dinger’s statement (in 1952) has been outspaced by reality: ‘. . . we never experiment with just one electron or atom or molecule. In thought experiments we sometimes assume we do, this invariably entails ridiculous consequences.’
Single molecule detection o ers the possibility of selecting, trapping, sorting, picking, and even manipulating molecules, especially biological macromolecules. Detection and spectroscopy of individual fluorescent molecules thus provide new tools not only in basic research but also in biotechnology and pharmaceutical industries (e.g. drug screening).

181 Introduction
1.7
Bibliography
Pioneering books
Bowen E. J. and Wokes F. (1953) Fluorescence of Solutions, Longmans, Green and Co., London.
Curie M. (1934) Luminescence des Corps Solides, Presses Universitaires de France, Paris.
Curie M. (1946) Fluorescence et Phosphorescence, Hermann, Paris.
Dake H. C. and De Ment J. (1941) Fluorescent Light and its Applications, Chemical Publishing Co., New York.
De Ment J. (1945) Fluorochemistry. A comprehensive Study Embracing the Theory and Applications of Luminescence and Radiation in Physicochemical Science, Chemical Publishing Co., New York.
Fo¨rster T. (1951) Fluoreszenz organischer Verbindungen, Vandenhoeck and Ruprecht, Go¨ttingen.
Hirschlaff E. (1939) Fluorescence and Phosphorescence, Chemical Publishing Co., New York.
Perrin F. (1931) Fluorescence. Duree´ Elementaire´ d’Emission Lumineuse, Hermann, Paris.
Pringsheim P. (1921, 1923, 1928) Fluorescenz und Phosphorescenz im Lichte der neueren Atomtheorie, Verlag von Julius Springer, Berlin.
Pringsheim P. (1949) Fluorescence and Phosphorescence, Interscience, New York.
Pringsheim P. and Vogel M. (1943)
Luminescence of Liquids and Solids and its Practical Applications, Interscience, New York.
Monographs and edited books after 1960
Baeyens W. R. G, de Keukeleire D. and Korkidis K. (Eds) (1991) Luminescence Techniques in Chemical and Biochemical Analysis, Marcel Dekker, New York.
Becker R. S. (1969) Theory and Interpretation of Fluorescence and Phosphorescence, Wiley Interscience, New York.
Beddard G. S. and West M. A. (Eds) (1981) Fluorescent Probes, Academic Press, London.
Berlman I. B. (1965, 1971) Handbook of Fluorescence Spectra of Aromatic Molecules, Academic Press, New York.
Birks J. B. (1970) Photophysics of Aromatic Molecules, Wiley-Interscience, London.
Birks J. B. (Ed.) (1975) Organic Molecular Photophysics, Vols. 1 and 2, John Wiley & Sons, London.
Bowen E. J. (Ed.) (1968) Luminescence in
Chemistry, Van Nostrand, London. Chen R. F. and Edelhoch H. (Eds) (1975,
1976) Biochemical Fluorescence. Concepts, Vols. 1 and 2, Marcel Dekker, New York.
Cundall R. B. and Dale R. E. (Eds) (1983)
Time-Resolved Fluorescence Spectroscopy in Biochemistry and Biology, Plenum Press, New York.
Czarnik A. W. (Ed.) (1992) Fluorescence Chemosensors for Ion and Molecule Recognition, American Chemical Society, Washington.
Demas J. N. (1983) Excited State Lifetime Measurement, Academic Press, New York.
Desvergne J.-P. and Czarnik A. W. (Eds)
(1997 ) Chemosensors of Ion and Molecule Recognition, Kluwer Academic Publishers, Dordrecht.
Dewey G. (Ed.) (1991) Biophysical and
Biochemical Aspects of Fluorescence Spectroscopy, Plenum Press, New York.
Galanin M. D. (1996) Luminescence of
Molecules and Crystals, Cambridge
International Science Publishing, Cambridge.
Goldberg M. C. (Ed.) (1989) Luminescence Applications in Biological, Chemical, Environmental, and Hydrological Sciences, American Chemical Society, Washington.
Guilbault G. (Ed.) (1973, 1990) Practical Fluorescence, Marcel Dekker, New York (1st edn: 1973; 2nd edn: 1990).
Guillet J. E. (1985) Polymer Photophysics and Photochemistry, Cambridge University Press, Cambridge, UK.
Hercules D. M. (Ed.) (1966) Fluorescence and Phosphorescence Analysis, Wiley Interscience, New York.
Jameson D. M. and Reinhart G. D. (Eds)
|
1.7 Bibliography |
19 |
|
|
|
(1989) Fluorescent Biomolecules, Plenum |
Rabek J. F. (Ed.) (1990) Photochemistry and |
|
Press, New York. |
Photophysics, CRC Press, Boca Raton. |
|
Krasovitskii B. M. and Bolotin B. M. (1988) |
Ramamurthy V. (Ed.) (1991) Photochemistry in |
|
Organic Luminescent Materials, VCH, |
Organized and Constrained Media, VCH, |
|
Weinheim. |
Weinheim. |
|
Lakowicz J. R. (1983, 1999) Principles of |
Rendell D. and Mowthorpe D. (Eds) (1987) |
|
Fluorescence Spectroscopy, Plenum Press, |
Fluorescence and Phosphorescence, John Wiley |
|
New York (1st edn, 1983; 2nd edn, 1999). |
and Sons, Chichester. |
|
Lakowicz J. R. (Ed.) Topics in Fluorescence |
Rettig W., Strehmel B., Schrader S. and |
|
Spectroscopy, Plenum Press, New York. Vol. |
Seifert H. (Eds) (1999) Applied Fluorescence |
|
1: Techniques (1991); Vol. 2: Principles |
in Chemistry, Biology and Medicine, Springer, |
|
(1991); Vol. 3: Biochemical Applications |
Berlin. |
|
(1992); Vol. 4: Probe Design and Chemical |
Schenk G. H. (1973) Absorption of Light and |
|
Sensing (1994); Vol. 5: Non-Linear and Two- |
Ultraviolet Radiation. Fluorescence and |
|
Photon-Induced Fluorescence (1997); Vol. 6: |
Phosphorescence Emission, Allyn and Bacon, |
|
Protein Fluorescence (2000). |
Boston. |
|
Lansing Taylor D., Waggoner A. S., Lanni |
Schulman S. G. (1977) Fluorescence and |
|
F., Murphy R. F. and Birge R. R. (Eds) |
Phosphorescence Spectroscopy: Physicochemical |
|
(1986) Applications of Fluorescence in the |
Principles and Practice, Pergamon Press, |
|
Biomedical Sciences, Alan R. Liss, New York. |
Oxford. |
|
Mielenz K. D. (Ed.) (1982) Measurement of |
Schulman S. G. (Ed.) Molecular Luminescence |
|
Photoluminescence, Academic Press, |
Spectroscopy, John Wiley and Sons, New |
|
Washington. |
York, Part 1 (1985); Part 2 (1988); Part 3 |
|
Mielenz K. D., Velapodi R. A. and |
(1993). |
|
Mavrodineanu R. (Eds) (1977) |
Slavik J. (Ed.) (1996) Fluorescence Microscopy |
|
Standardization in Spectrometry and |
and Fluorescent Probes, Plenum Press, New |
|
Luminescence Measurement, U.S. Dept. |
York. |
|
Commerce, New York. |
Suppan P. (1994) Chemistry and Light, Royal |
|
Miller J. N. (Ed.) (1981) Standards in |
Society of Chemistry, Cambridge. |
|
Fluorescence Spectrometry, Ultraviolet |
Turro N. J. (1978) Modern Molecular |
|
Spectrometry Group, Chappman and Hall, |
Photochemistry, The Benjamin/Cummings |
|
London. |
Publishing Co., Menlo Park. |
|
Murov S. L., Carmichael I. and Hug G. L. |
Udenfriend S., Fluorescence Assay in Biology |
|
(1993) Handbook of Photochemistry, 2nd edn, |
and Medecine, Academic Press, New York, |
|
Marcel Dekker, New York. |
Vol. 1 (1962); Vol. 2 (1971). |
|
O’Connor D. V. and Phillips D. (1984) Time- |
Valeur B. and Brochon J. C. (Eds) (2001) |
|
Correlated Single Photon Counting, Academic |
New Trends in Fluorescence Spectroscopy. |
|
Press, London. |
Applications to Chemical and Life Sciences, |
|
Parker C. A. (1968) Photoluminescence of |
Springer-Verlag, Berlin. |
|
Solutions, Elsevier, Amsterdam. |
Wolfbeis O. S. (Ed.) (1993) Fluorescence |
|
Pesce A. J., Rosen C.-G. and Pasby T. L. (Eds) |
Spectroscopy. New Methods and |
|
(1971) Fluorescence Spectroscopy, Marcel |
Applications, Springer-Verlag, Berlin. |
|
Dekker, New York. |
|
|

Molecular Fluorescence: Principles and Applications. Bernard Valeur
> 2001 Wiley-VCH Verlag GmbH
ISBNs: 3-527-29919-X (Hardcover); 3-527-60024-8 (Electronic)
20
2
Absorption of UV–visible light
La lumie`re (. . .) donne la couleur et l’e´clat a` toutes les productions de la nature et de l’art; elle multiplie l’univers en le peignant dans les yeux de tout ce qui respire.
Abbe´ Nollet, 1783
[Light (. . .) gives color and brilliance to all works of nature and of art; it multiplies the universe by painting it in the eyes of all that breathe.]
The aim of this chapter is to recall the basic principles of light absorption by molecules. The reader is referred to more specialized books for further details.
2.1
Types of electronic transitions in polyatomic molecules
An electronic transition consists of the promotion of an electron from an orbital of a molecule in the ground state to an unoccupied orbital by absorption of a photon. The molecule is then said to be in an excited state. Let us recall first the various types of molecular orbitals.
As orbital can be formed either from two s atomic orbitals, or from one s and one p atomic orbital, or from two p atomic orbitals having a collinear axis of symmetry. The bond formed in this way is called a s bond. A p orbital is formed from two p atomic orbitals overlapping laterally. The resulting bond is called a p bond. For example in ethylene (CH2 bCH2), the two carbon atoms are linked by one s and one p bond. Absorption of a photon of appropriate energy can promote one of the p electrons to an antibonding orbital denoted by p . The transition is then called p ! p . The promotion of a s electron requires a much higher energy (absorption in the far UV) and will not be considered here.
Amolecule may also possess non-bonding electrons located on heteroatoms such as oxygen or nitrogen. The corresponding molecular orbitals are called n or-
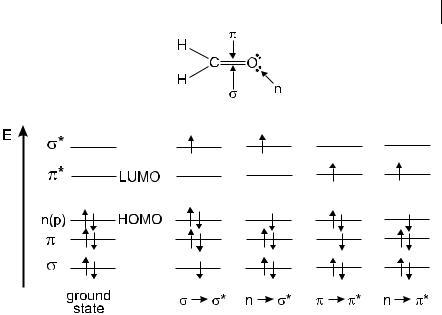
2.1 Types of electronic transitions in polyatomic molecules 21
Fig. 2.1. Energy levels of molecular orbitals in formaldehyde (HOMO: Highest Occupied Molecular Orbitals; LUMO: Lowest Unoccupied Molecular Orbitals) and possible electronic transitions.
bitals. Promotion of a non-bonding electron to an antibonding orbital is possible and the associated transition is denoted by n ! p .
The energy of these electronic transitions is generally in the following order:
n ! p < p ! p < n ! s < s ! p < s ! s
To illustrate these energy levels, Figure 2.1 shows formaldehyde as an example, with all the possible transitions. The n ! p transition deserves further attention: upon excitation, an electron is removed from the oxygen atom and goes into the p orbital localized half on the carbon atom and half on the oxygen atom. The n–p excited state thus has a charge transfer character, as shown by an increase in the dipole moment of about 2 D with respect to the ground state dipole moment of CbO (3 D).
In absorption and fluorescence spectroscopy, two important types of orbitals are considered: the Highest Occupied Molecular Orbitals (HOMO) and the Lowest Unoccupied Molecular Orbitals (LUMO). Both of these refer to the ground state of the molecule. For instance, in formaldehyde, the HOMO is the n orbital and the LUMO is the p orbital (see Figure 2.1).
When one of the two electrons of opposite spins (belonging to a molecular orbital of a molecule in the ground state) is promoted to a molecular orbital of higher energy, its spin is in principle unchanged (Section 2.3) so that the total spin quantum number (S ¼ Ssi, with si ¼ þ 12 or 12) remains equal to zero. Because the multi-
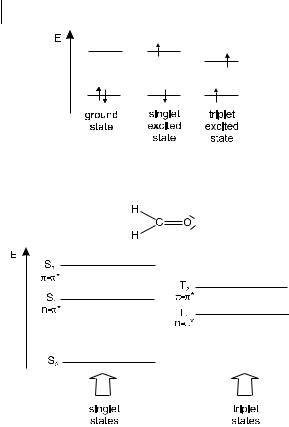
22 2 Absorption of UV–visible light
Fig. 2.2. Distinction between singlet and triplet states, using formaldehyde as an example.
plicities of both the ground and excited states ðM ¼ 2S þ 1Þ is equal to 1, both are called singlet state (usually denoted S0 for the ground state, and S1; S2; . . . for the excited states) (Figure 2.2)1). The corresponding transition is called a singlet–singlet transition. It will be shown later that a molecule in a singlet excited state may undergo conversion into a state where the promoted electron has changed its spin; because there are then two electrons with parallel spins, the total spin quantum number is 1 and the multiplicity is 3. Such a state is called a triplet state because it corresponds to three states of equal energy. According to Hund’s Rule, the triplet state has a lower energy than that of the singlet state of the same configuration.
In a molecule such as formaldehyde, the bonding and non-bonding orbitals are localized (like the bonds) between pairs of atoms. Such a picture of localized orbitals is valid for the s orbitals of single bonds and for the p orbitals of isolated double bonds, but it is no longer adequate in the case of alternate single and double carbon–carbon bonds (in so-called conjugated systems). In fact, overlap of the p orbitals allows the electrons to be delocalized over the whole system (resonance
1)In some cases, the ground state is not a singlet state, e.g. dioxygen, anion and cation radicals of aromatic molecules.