
Therapeutic Micro-Nano Technology BioMEMs - Tejlal Desai & Sangeeta Bhatia
.pdf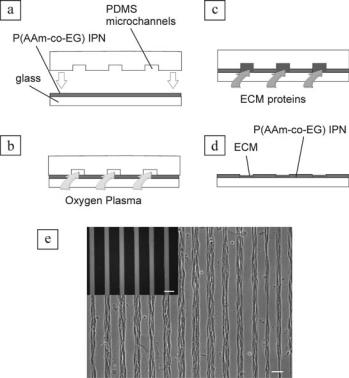
358 |
ALBERT FOLCH AND ANNA TOUROVSKAIA |
FIGURE 19.7. Schematic illustration (a–d) of the procedure for cellular micropatterning based on the use of PDMS microchannels as masks for selective surface etching [4]: (a) The PDMS mold is placed onto a glass substrate homogeneously grafted with the P(AAm-co-EG) interpenetrating polymer network (IPN); (b) Selective etching: the IPN is selectively removed after exposure to oxygen plasma, which leaves alternating lines of IPN-free glass and IPN; (c) Selective deposition: the microchannels are filled with extracellular matrix (ECM) protein to promote cell attachment. After flushing with PBS, the microchannels are removed, leaving lines of ECM protein surrounded by IPN. The ECM protein/IPN patterned glass is incubated with a cell suspension and unattached cells are removed by exchanging the medium; (e) Phase-contrast image of NIH 3T3 fibroblasts attached to the lines of fibronectin conjugated to Alexa Fluor 488 dye. Scale bars are 100 μm.
bare glass; the bare glass areas are amenable to protein adsorption and cell attachment using traditional cell seeding protocols. This method allows for producing cellular micropatterns with isolated or connected features, with sub-cellular resolution, and containing more than one cell type. The cellular micropatterns exhibit excellent temporal stability over periods of time longer than two weeks even when serum is present in the culture medium and at high cell densities. Other examples of PEG IPN patterns can be seen in Fig. 19.6 (top right pattern reading “UW” and bottom left image of myotubes in a microchannel). The PEG IPN surface chemistry is specially important in our long-term muscle cell differentiation studies; muscle cells are seeded at low densities on lanes of fibronectin (physisorbed on glass) surrounded by PEG IPN and, over the following days, the cells proliferate until they reach confluence. Importantly, after cell division the daughter cells also stay confined to the fibronectin lines and finally achieve confluence and fuse, forming a “myotube”. The process of division until contact and fusion occur is paralleled in non-microfluidic,
CELL BIOLOGY ON A CHIP |
359 |
non-micropatterned cultures; however, in non-micropatterned cultures the myotubes form at seemingly random orientations rather than at the orientation dictated by the surface chemistry.
19.4.3. From Cells in Large Static Volumes to Cells in Small Flowing Volumes
As pointed out above, in high-density microfluidic cell cultures the microenvironment’s nutrients, pH and gas concentrations cannot be kept constant for long periods of time, and replenishing the volume of the microfluidic chamber becomes necessary. An extreme deviation of a “proper” cell culture environment results in obvious cell death. However, the effects can be much subtler, due to the fact that a) cells are exposed to shear stress from the flow, and/or b) at the cell membrane surface, the actual concentration of growth factors (that either bind to cells or are secreted by them) “seen” by the cell depends on the speed of the flow, which effectively “washes away” the growth factor molecules that happen to diffuse into the stream. In our studies of muscle cell fusion in microfluidic environments (Fig. 19.4), we have indeed observed that muscle cell differentiation (as ascertained by the ability of cells to fuse) proceeds at different paces depending on the flow rates; at high flow rates, cell fusion takes longer to occur, likely due to the dilution of cell-secreted growth factors into the flow.
19.4.4. From a Homogeneous Bath to Microfluidic Delivery of Biochemical Factors
In traditional cell culture, cells are bathed in a homogeneous medium; thus, any cellular response that relies on graded or focal exposure of the cell to a given factor will not be observable, including many cell growth and motility phenomena. Traditionally, focal stimulation of cells with fluids has been possible only using micropipettes, which eject fluid upon application of a pressure pulse (“puffing”) [104] or voltage pulse (“iontophoresis”) [104–106], or using caged compounds that are uncaged by a laser pulse [107]. Clearly, these techniques result in very low throughputs and/or poorly-characterized volumes or gradients, require bulky equipment and substantial manual skill, and are unscalable (stimulation at more than 3 or 4 sites is not practical). Microfluidic systems, on the other hand, constitute a technology for directing many different fluids to many different (small) cell populations [39, 41, 108] that is scalable, amenable to fluid dynamics modeling [109], and where the delivery system is pre-aligned with the cells. Takayama et al. first demonstrated heterogeneous labeling of internal cellular structures and subcellular enzymatic treatment using laminar flows [39, 41, 108] in microchannels. In our lab, we have exposed microengineered myotubes to a heterogeneous flow consisting of a fluorescently-labeled bungarotoxin (BTX) stream sided by two BTX-free streams; PEG-IPN patterns were defined at the floor of the microchannel to produce (after seeding and culturing muscle cells for several days in the microchannel) myotubes perpendicular to the direction of the flow. As shown in Fig. 19.8, only the central portions of the myotubes were actually labeled with BTX. Thus, with microfluidic systems it is possible to confine a membrane receptor-labeling assay to a region smaller than a cell; importantly, the fact that the assay is subcellular allows for using lethal, high-affinity neurotoxin labels like BTX. This type of subcellular labeling should allow for the live tracking of a variety of membrane proteins.
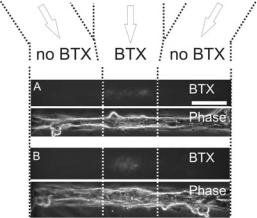
360 |
ALBERT FOLCH AND ANNA TOUROVSKAIA |
FIGURE 19.8. Acetylcholine receptor (AChR) clusters visualized by binding of a-bungarotoxin conjugated with Alexa Fluor 488 dye (BTX). The labeling was confined to a central portion of the myotubes (between the white dotted lines) and the myotubes span the entire microchannel. Each pair of BTX/Phase images (labeled A and B) correspond to the same myotube, with A being upstream of B; the images labeled “Phase” are phase-contrast images. The scale bar is 100 μm.
19.5. CONCLUSION
In sum, following a trend similar to the technology forces that pressed for the miniaturization of electronic devices, there is a growing interest in “going micro” in cell culture technology. First and foremost, microfluidic cell culture systems enable the modulation of signaling factors on a scale ranging millimiters to sub-cellular dimensions, thus providing for a more reproducible in-vivo environment and allowing for a new class of experiments that require heterogeneous and/or local stimulation of cells. For the same price, microfluidic cultures are also attractive in that 1) by virtue of being batch-fabricated, they allow for addressing large numbers of “units” (either cells, groups of cells, microchambers, etc.) at high-throughput and at low cost, and 2) by virtue of their size, they consume small reagent volumes and require small numbers of cells compared to traditional macrofluidic cultures.
REFERENCES
[1]A. Folch, B.-H. Jo, D. Beebe, and M. Toner. J. Biomed. Mater. Res., 52:346, 2000.
[2]R.G. Harrison. Proc. Soc. Exp. Biol. Med., 4:140, 1907.
[3]A. Carrel. J. Exp. Med., 15:516, 1912.
[4]A. Tourovskaia, T. Barber, B.T. Wickes, D. Hirdes, B. Grin, D.G. Castner, K.E. Healy, and A. Folch. Langmuir, 19:4754, 2003.
[5]R.I. Freshney. Culture of Animal Cells: A Manual of Basic Technique, (4th ed.). Wiley-Liss, New York, 2000.
[6]R.F. Service. Science, 282:396, 1998,
[7]P. Gravesen, J. Branebjerg, and O.S. Jensen. J. Micromech. Microeng., 3:168, 1993.
[8]J.P. Brody, P. Yager, R.E. Goldstein, and R.H. Austin. Biophys. J., 71:3430, 1996.
[9]S.C. Jacobson, R. Hergenroder, L.B. Koutny, and J.M. Ramsey. Anal. Chem., 66:1114, 1994.
CELL BIOLOGY ON A CHIP |
361 |
[10]C.S. Effenhauser, G.J. M. Bruin, A. Paulus, and M. Ehrat. Anal. Chem., 69:3451, 1997.
[11]R.M. McCormick, R.J. Nelson, M.G. Alonso-Amigo, D.J. Benvegnu, and H.H. Hooper. Anal. Chem., 69:2626, 1997.
[12]G.H.W. Sanders and A. Manz. Trac-Trends Anal. Chem., 19:364, 2000.
[13]M.A. Shoffner, J. Cheng, G.E. Hvichia, L.J. Kricka, and P. Wilding. Nucleic Acids Res., 24:375, 1996.
[14]A.T. Woolley, D. Hadley, P. Landre, A.J. deMello, R.A. Mathies, and M.A. Northrup. Anal. Chem. 68:4081, 1996.
[15]D.T. Burke, M.A. Burns, and C. Mastrangelo. Genome Res., 7:189, 1997.
[16]M.U. Kopp, A.J. Mello, and A. Manz. Science, 280:1046, 1998.
[17]Z.H.Fan, S. Mangru, R. Granzow, P. Heaney, W. Ho, Q.P. Dong, and R. Kumar. Anal. Chem., 71:4851, 1999.
[18]E. Altendorf, D. Zebert, M. Holl, A. Vannelli, C. Wu, and T. Schulte. In D. Harrison, and A. van den Berg (eds.). Micro Total Analysis Syst. ’98 , Kluwer Academic, Banff, Canada, p. 73, 1998.
[19]G. Ocvirk, H. Salimi-Moosavi, R.J. Szarka, E. Arriaga, P.E. Andersson, R. Smith, N.J. Dovichi, and D.J Harrison. In D. Harrison, and A. van den Berg (eds.). Micro Total Analysis Syst. ’98, Kluwer Academic, Banff,Canada, p. 203, 1998.
[20]Q. Xue, F. Foret, Y.M. Dunayevskiy, P.M. Zavracky, N.E. McGruer, and B.L. Karger. Anal. Chem., 69:426, 1997.
[21]R.D. Oleschuk and D.J. Harrison. Trac-Trends in Anal. Chem., 19:379, 2000.
[22]J.D. Trumbull, I.K. Glasgow, D.J. Beebe, and R.L. Magin. IEEE Trans. Biomed. Engin., 47:3, 2000.
[23]L.B. Koutny, D. Schmalzing, T.A. Taylor, and M. Fuchs. Anal. Chem., 68:18, 1996.
[24]N. Chiem and D.J. Harrison. Anal. Chem., 69:373, 1997.
[25]N.H. Chiem and D.J. Harrison. Clin. Chem., 44:591, 1998.
[26]A.E. Kamholz, B.H. Weigl, B.A. Finlayson, and P. Yager. Anal. Chem., 71:5340, 1999.
[27]B.H. Weigl and P. Yager. Sens. Actu. B-Chem., 39:452, 1997.
[28]B.H. Weigl and P. Yager. Science, 283:346, 1999.
[29]E.T. Lagally, P.C. Simpson, and R.A. Mathies. Sens. Actua. B-Chem., 63:138, 2000.
[30]L.C. Waters, S.C. Jacobson, N. Kroutchinina, J. Khandurina, R.S. Foote, and J.M. Ramsey. Anal. Chem., 70:158, 1998.
[31]A.T. Woolley, K. Lao, A.N. Glazer, and R.A. Mathies. Anal. Chem., 70:684, 1998.
[32]J. Cheng, E.L. Sheldon, L. Wu, A. Uribe, L.O. Gerrue, J. Carrino, M.J. Heller, and J.P. O’Connell. Nat. Biotech., 16:541, 1998.
[33]P.C. Li and D.J. Harrison. Anal. Chem., 69:1564, 1997.
[34]E. Altendorf, D. Zebert, M. Holl, and P. Yager. Proc. Int. Solid State Sensors and Actuators Conf. (Transducers 97), IEEE, Chicago, IL, U.S.A., p. 531, 1997.
[35]P.R.C. Gascoyne, W. Xiao-Bo, H. Ying, and F.F. Becker. IEEE Trans. Ind. Appl., 33:670, 1997.
[36]S.J. Choi, I. Glasgow, H. Zeringue, D.J. Beebe, and M.B. Wheeler. Biol. Reprod., 58:96, 1998.
[37]N. Sutton, M.C. Tracey, I.D. Johnston, R.S. Greenaway, and M.W. Rampling. Microvascular Res., 53:272, 1997.
[38]A. Folch, A. Ayon, O. Hurtado, M.A. Schmidt, and M. Toner. J. Biomech. Eng., 121:28, 1999.
[39]S. Takayama, J.C. McDonald, E. Ostuni, M.N. Liang, P.J.A. Kenis, R.F. Ismagilov, and G.M. Whitesides.
Proc. Natl. Acad. Sci. U.S.A., 96:5545, 1999.
[40]D.T. Chiu, N.L. Jeon, S. Huang, R.S. Kane, C.J.Wargo, I.S. Choi, D.E. Ingber, and G.M. Whitesides. Proc. Natl. Acad. Sci. U.S.A., 97:2408, 2000.
[41]S. Takayama, E. Ostuni, P. LeDuc, K. Naruse, D.E. Ingber, and G.M. Whitesides. Nature, 411:1016, 2001.
[42]Y. Xia, E. Kim, and G.M. Whitesides. J. Electrochem. Soc., 143:1070, 1996.
[43]J.C. McDonald, D.C. Duffy, J.R. Anderson, D.T. Chiu, H.K. Wu, O.J.A. Schueller, and G.M. Whitesides.
Electrophoresis, 21:27, 2000.
[44]S.K.W. Dertinger, D.T. Chiu, N.L. Jeon, and G.M. Whitesides. Anal. Chem., 73:1240, 2001.
[45]B.H. Jo, L.M. Van Lerberghe, K.M. Motsegood, and D.J. Beebe. J. Microelectromech. Syst., 9:76, 2000.
[46]J.R. Anderson, D.T. Chiu, R.J. Jackman, O. Cherniavskaya, J.C. McDonald, H.K.Wu, S.H. Whitesides, and G.M. Whitesides. Anal. Chem., 72:3158, 2000.
[47]R.F. Ismagilov, J.M.K. Ng, P.J.A. Kenis, and G.M. Whitesides. Anal. Chem., 73:5207, 2001.
[48]R.F. Ismagilov, D. Rosmarin, P.J. A.Kenis, D.T. Chiu, W. Zhang, H.A. Stone, and G.M. Whitesides. Anal.Chem., 73:4682, 2001.
362 |
ALBERT FOLCH AND ANNA TOUROVSKAIA |
[49]Y. Kikutani, H. Hisamoto, M. Tokeshi, and T. Kitamori. In J. M. Ramsey and A. v. d. Berg (eds.). Micro Total Analysis Systems. Kluwer Academic, Monterrey, CA, p. 161, 2001.
[50]H.-P. Chou, M.A. Unger, and S.R. Quake. Biomed. Microdev., 3:323, 2001.
[51]J. Liu, M. Enzelberger, and S. Quake. Electrophoresis, 23:1531, 2002.
[52]M.A. Unger, H.P. Chou, T. Thorsen, A. Scherer, and S.R. Quake. Science, 288:113, 2000.
[53]T. Thorsen, S.J. Maerkl, and S.R. Quake. Science, 298:580, 2002.
[54]V. Studer, G. Hang, A. Pandolfi, M. Ortiz, W.F. Anderson, and S.R. Quake. J Appl. Phys., 95:393, 2004.
[55]B.H. Jo, J. Moorthy, and D.J. Beebe. In v. d. B. et. al. (ed.), Micro Total Analysis Systems 2000 Kluwer Academic Publishers, p. 335, 2000.
[56]K. Hosokawa and R. Maeda. J. Micromechan. Microeng., 10:415, 2000.
[57]E. Neher. Science, 256:498, 1992.
[58]N. Fertig, R.H. Blick, and J.C. Behrends. Biophys. J., 82:3056, 2002.
[59]N. Fertig, M. Klau, M. George, R.H. Blick, and J.C. Behrends. Appl. Phys. Lett., 81:4865, 2002.
[60]K.G. Klemic, J.F. Klemic, M.A. Reed, and F.J. Sigworth. Biosen. Bioelectron., 17:597, 2002.
[61]T. Lehnert, M.A.M. Gijs, R. Netzer, and U. Bischoff. Appl. Phys. Lett., 81:5063, 2002.
[62]C.G. Galbraith and M.P. Sheetz. J. Cell Biol., 147:1313, 1999.
[63]R. Pethig. Crit. Rev. Biotechnol., 16:331, 1996.
[64]E. Richter, G. Fuhr, T. Muller, S. Shirley, S. Rogaschewski, K. Reimer, and C. Dell. J. Mater. Science-Mater. Med., 7:85, 1996.
[65]T. Matsue, N. Matsumoto, and I. Uchida. Electrochim. Acta, 42:3251, 1997.
[66]J. Voldman, R.A. Braff, M. Toner, M.L. Gray, and M.A. Schmidt. Biophys. J., 80:531, 2001.
[67]J. Voldman, M.L. Gray, M. Toner, and M.A. Schmidt. Anal. Chem., 74:3984, 2002.
[68]A. Folch and M. Toner. Ann. Rev. Biomed. Eng., 2:227, 2000.
[69]R.G. Harrison. Anat. Rec., 6:181, 1912.
[70]P. Weiss. J. Exp. Zool., 100:353, 1945.
[71]A.S.G. Curtis and M. Valverde. J. Natl. Cancer Inst., 33:15, 1964.
[72]Y.A. Rovensky, I.L. Slavnaja, and J.M. Vasiliev. Exp. Cell Res., 65:193, 1971.
[73]E.J. Furshpan, P.R. MacLeish, P.H.O’Lague, and D.D. Potter. Proc. Natl. Acad. Sci. U.S.A., 73:4225, 1976.
[74]S. Grumbacher-Reinert. Proc. Natl. Acad. Sci. U.S.A., 86:7270, 1989.
[75]D.C. Turner, J. Lawton, P. Dollenmeier, R. Ehrismann, and M. Chiquet. Dev. Biol., 95:497, 1983.
[76]M. Lieberman, A.E. Roggeveen, J.E. Purdy, and E.A. Johnson. Science, 175:909, 1972.
[77]O.Y. Ivanova and L.B. Margolis. Nature, 242:200, 1973.
[78]R.A. Rovasio, A. Delouvee, K.M. Yamada, R. Timpl, and J.P. Thiery. J. Cell. Biol., 96:462, 1983.
[79]R. Singhvi, A. Kumar, G.P. Lopez, G.N. Stephanopoulos, D.I. Wang, G.M.Whitesides, and D.E. Ingber. Science, 264:696, 1994.
[80]C.S. Chen, M. Mrksich, S. Huang, G.M. Whitesides, and D.E. Ingber. Science, 276:1425, 1997.
[81]C.H. Thomas, J.B. Lhoest, D.G. Castner, C.D. McFarland, and K.E. Healy. J. Biomech. Eng., 121:40, 1999.
[82]S.N. Bhatia, M.L. Yarmush, and M. Toner. J. Biomed. Mater. Res., 34:189, 1997.
[83]S.N. Bhatia, U.J. Balis, M.L. Yarmush, and M. Toner. Biotechnol. Prog., 14:378, 1998.
[84]Y.N. Xia and G.M. Whitesides. Angew. Chem.-Int. Edit. Engl., 37:551, 1998.
[85]A. Folch, S. Mezzour, M. During, O. Hurtado, M. Toner, and R. Mueller. Biomed. Microdev., 2:207, 2000.
[86]V.A. Liu and S.N. Bhatia. Biomed. Microdev., 4:257, 2002.
[87]S. Petronis, K.L. Eckert, J. Gold, and E. Wintermantel. J. Mater. Sci.-Mater. Med., 12:523, 2001.
[88]G.D. Pins, M. Toner, and J.R. Morgan. FASEB J., 14:593, 2000.
[89]J. Ziauddin and D.M. Sabatini. Nature, 411:107, 2001.
[90]H.M. McConnell, J.C. Owicki, J.W. Parce, D.L. Miller, G.T. Baxter, H.G.Wada, and S. Pitchford. Science, 257:1906, 1992.
[91]L. Bousse and W. Parce. IEEE Eng. Med. Biol. Mag., 13:396, 1994.
[92]P. Fromherz and A. Stett. Phys. Rev. Lett., 75:1670, 1995.
[93]A. Folch and M. Toner. Biotechnol. Prog., 14:388, 1998.
[94]V.A. Liu, W.E. Jastromb, and S.N. Bhatia. J. Biomed. Mater. Res., 60:126, 2002.
[95]M. Mrksich, L.E. Dike, J. Tien, D.E. Ingber, and G.M. Whitesides. Exp. Cell Res., 235:305, 1997.
[96]E. Ostuni, R. Kane, C.S. Chen, D.E. Ingber, and G.M. Whitesides. Langmuir, 16:7811, 2000.
[97]P.D. Drumheller and J.A. Hubbell. In J. D. Bronzino (ed.) The Biomedical Engineering Handbook. CRCPress, Boca Raton, FL, p. 1583, 1995.
CELL BIOLOGY ON A CHIP |
363 |
[98]M. Mrksich. Cell. Mol. Life Sci., 54:653, 1998.
[99]N. Patel, R. Padera, G.H.W. Sanders, S.M. Cannizzaro, M.C. Davies, R. Langer, C.J. Roberts, S.J.B. Tendler, P.M. Williams, and K.M. Shakesheff. FASEB J. 12:1447, 1998.
[100]C.B. Herbert, T.L. McLernon, C.L. Hypolite, D.N. Adams, L. Pikus, C.C. Huang, G.B. Fields, P.C. Letourneau, M.D. Distefano, and W.S. Hu. Chem. Biol., 4:731, 1997.
[101]J.P. Bearinger, D.G. Castner, S.L. Golledge, A. Rezania, S. Hubchak, and K.E.Healy. Langmuir, 13:5175, 1997.
[102]J.P. Bearinger and K.E. Healy. J. Dent. Res., 76:2948, 1997.
[103]S. Saneinejad and M.S. Shoichet. J. Biomed. Mater. Res. 42:13, 1998.
[104]T.W. Stone. Microiontophoresis and Pressure Ejection, Vol. 8, (1st Ed.). Wiley, John & Sons, Inc., 1985.
[105]W.L. Nastuk. J. Cell. Comp. Physiol., 42:249, 1953.
[106]J. del Castillo and B. Katz. J. Physiol., 128:157, 1955.
[107]K. Kandler, L.C. Katz, and J.A. Kauer. Nat. Neurosci., 1:119, 1998.
[108]S. Takayama, E. Ostuni, P. LeDuc, K. Naruse, D.E. Ingber, and G.M. Whitesides. Chem. Biol., 10:123, 2003.
[109]G.A. Ledezma, A. Folch, S.N. Bhatia, M.L. Yarmush, and M. Toner, J. Biomech. Eng., 121:58, 1999.
About the Editors
Professor Mauro Ferrari is a pioneer in the fields of bioMEMS and biomedical nanotechnology. As a leading academic, a dedicated entrepreneur, and a vision setter for the Nation’s premier Federal programs in nanomedicine, he brings a three-fold vantage perspective to his roles as Editor-in-Chief for this work. Dr. Ferrari has authored or co-authored over 150 scientific publications, 6 books, and over 20 US and International patents. Dr. Ferrari is also Editor-in-Chief of Biomedical Microdevices and series editor of the new Springer series on Emerging Biomedical Technologies.
Several private sector companies originated from his laboratories at the Ohio State University and the University of California at Berkeley over the years. On a Federal assignment as Special Expert in Nanotechnology and Eminent Scholar, he has provided the scientific leadership for the development of the Alliance for Cancer Nanotechnology of the National Cancer Institute, the world-largest medical nanotechnology operation to date. Dr. Ferrari trained in mathematical physics in Italy, obtained his Master’s and Ph.D. in Mechanical Engineering at Berkeley, attended medical school at The Ohio State University, and served in faculty positions in Materials Science and Engineering, and Civil and Environmental Engineering in Berkeley, where he was first tenured. At Ohio State he currently serves as Professor of Internal Medicine, Division of Hematology and Oncology, as Edgar Hendrickson Professor of Biomedical Engineering, and as Professor of Mechanical Engineering. He is Associate Director of the Dorothy M. Davis Heart and Lung Research Institute, and the University’s Associate Vice President for Health Science, Technology and Commercialization.
Dr. Tejal Desai is currently an Associate Professor of Physiology and Bioengineering at the University of California, San Francisco. She is also a member of the California Institute for Quantitative Biomedical Research and the UCSF/UCB Bioengineering Graduate Group. Prior to joining UCSF, she was a professor of Biomedical Engineering at Boston University and Associate Director of the Center for Nanoscience and Nanobiotechnology at BU. She received the Sc.B. degree in Biomedical Engineering from Brown University (Providence, RI) in 1994 and the Ph.D. degree in bioengineering from the joint graduate program at University of California, Berkeley and the University of California, San Francisco, in 1998. Dr. Tejal Desai directs the Laboratory of Therapeutic Micro and Nanotechnology. In addition to authoring over 60 technical papers, she is presently an associate editor of Langmuir, Biomedical Microdevices, and Sensors Letters and is editing a book on Therapeutic Microtechnology. She has chaired and organized several conferences and symposia
366 |
ABOUT THE EDITORS |
in the area of bioMEMS, micro and nanofabricated biomaterials, and micro/nanoscale tissue engineering.
Desai’s research efforts have earned her numerous awards. In 1999, she was recognized by Crain’s Chicago Business magazine with their annual “40 Under 40” award for leadership. She was also named that year by Technology Review Magazine as one of the nation’s “Top 100 Young Innovators” and Popular Science’s Brilliant 10. Desai’s teaching efforts were recognized when she won the College of Engineering Best Advisor/Teacher Award. She also won the National Science Foundation’s “New Century Scholar” award and the NSF Faculty Early Career Development Program “CAREER” award, which recognizes teacher-scholars most likely to become the academic leaders of the 21st century. Her research in therapeutic microtechnology has also earned her the Visionary Science Award from the International Society of BioMEMS and Nanotechnology in 2001, a World Technology Award Finalist in 2004, and the EURAND award for innovative advances in drug delivery.
Sangeeta N. Bhatia, M.D., Ph.D. is an Associate Professor at the Massachusetts Institute of Technology. Her work focuses on using microand nanotechnology tools to repair damaged tissues. Dr. Bhatia trained at Brown, MIT, and Harvard. After postdoctoral training at the Massachusetts General Hospital, she was a member of the Bioengineering Department at University of California at San Diego for 6 years. In 2005, she returned to Boston to join the MIT faculty. She has been awarded the David and Lucile Packard Fellowship given to ‘the nation’s most promising young professors in science and engineering,’ the MIT TR100 Young Innovators Award, and been named one of San Diego’s ‘50 People to Watch in 2004’. Her research portfolio includes funding from NIH, NSF, DARPA, NASA, the Whitaker Foundation, the Packard Foundation, and private industry. She co-authored the first undergraduate textbook on tissue engineering and is a frequent advisor to governmental organizations on cell-based sensing, nanobiotechnology, and tissue engineering. She holds 12 issued or pending patents and has worked in industry at Pfizer, Genetics Institute, ICI Pharmaceuticals, and Organogenesis.
Index
adhesive-mediated fabrication, 28–29 pressure-assisted microsyringe, 29 three-dimensional printing, 28–29
aimine functionalization, 249 air-sheath flow cytometry, 69–71 Alzheimer’s disease, 172 angiogenesis, 334
assays. See also cell function, advanced microfluidic assays for; immunoassays
surface-based, 147 asthma, 194, 197, 206 avidin immobilization, 251
axonal outgrowth promotion in CNS/PNS, 4–6 inhibitory effects alleviation, 6
response after injury, 4
substrates to support growing axons, 5 trophic factors to stimulate, 5
bacteriophage, for in vivo phage screening, 128–129
bioadhesive drug delivery system, 238–240 bioartificial tissues, 326
biocompatible quantum dots (QDs), 137–152 applications, 146–152
cell labeling/tracking experiments, 149–150 in vitro immunoassays/nanosensors, 146–149 in vivo live animal imaging, 150–152
future work, 152 introduction, 137–138 optical properties, 142–146
comparative photobleaching curve, 145–146 single QD imaging, 145
synthesis/surface chemistry, 138–142 surface chemistry modification, 141–142
synthesis of QDs soluble in organic solvents, 138–141
bioluminescent proteins, 84–85
biomaterials. See also peptide nanobiomaterials photonic crystals, 217
tailor-made, 39, 51–52 top-down approach, 39
biosensing, multi-phenotypic cellular arrays for, 79–90
array examples, 87–88 array fabrication, 81–83
photolithography, 81–82 poly(ethylene) glycol hydrogels, 83 soft lithography, 82–83
surface patterning, 81 cell-based sensor detection, 84–87
gene expression/protein up-regulation, 84–85 bioluminescent proteins, 84–85
green fluorescent, 85
intracellular fluorescent probes, 86–87 microelectronics, 84
future work, 88–90 introduction, 79–81
bovine serum albumin, 273–275 brain-derived neuotrophic factor (BDNF), 5 brain tumors, 101–103
breast cancer, 130
Caco-2 in vitro studies, 255, 257 cadherin adhesions, 329
cAMP, 6
cancer, 167, 194, 203 cancer treatment, 165–168 cell adhesion, 325–340
bioartificial tissues, 326 conclusions, 339
controlling cell-environment interaction, 330–337
with defined surface chemistries, 331–332 examples of patterning-based studies
on cell-matrix interactions, 336–337 on cell-to-cell interactions, 333–334
with surface patterning, 332–334 future work, 337–339
cell positioning, 338–339 3-D patterning, 338
new materials, 337
substrate mechanics patterning, 339
368
cell adhesion (cont.) introduction, 325–327 microfabricated cells, 326
regulating cell function via adhesive microenvironment, 327–330
cell-based combinational screening, 349
cell biology on a chip. See cell culture, microfluidic cell culture, microfluidic, 345–360
conclusion, 360
experimentation throughput devices, 347–354 equipment change to “chip-cubators,” 349 fluid metering, 351–354
scaled down volume of needed cells, 349–350 serial pipetting/highly parallel micromixers, 347,
349
single-cell probing/manipulation, 354 smaller volumes of reagents, 349–350
introduction, 345–346
central elements/limitations of, 348 circumventing limitations of, 346–347 multi-signal changing environment of cells, 345 in vivo vs. in vitro systems, 345–346
microevironment control devices, 354-360 biochemical factors delivery, 359–360 cells in small flowing volumes, 359 cellular micropatterning methods, 355–356 cellular micropatterns, 356
substrates, 356–359
cell function, advanced microfluidic assays for, 55–75 conclusion, 75
examples, 61–75
air-sheath flow cytometry, 69–71 immunoassay, 71–72
microfluidic, 73–74 T-sensor device for, 72
microscale integrated sperm sorter, 68–69 multiple laminar streams, 63–65 PARTCELL, 66–68
patterning with individual microfluidic channels, 61–63
introduction, 55–56 microfabrication, 56–58
soft lithography PDMS structures, 57–58 microscale phenomena, 58–61
laminar flow, 59–60 scaling effects, 59 surface tension, 60–61
cell proliferation, 336
cell replacement. See islet cell replacement celluar arrays. See biosensing, multi-phenotypic
cellular arrays for cellular transduction, 66
central nervous system (CNS). See neural regeneration chondrocytes, 325
CNS (central nervous system), 3
INDEX
collagen, 185, 339
collagen, as growth permissive scaffold for nerve generation, 9–10
coloror fluorescentcontrast agencies, 201 communicating hydrocephalus, 99
controllable reservoir-based drug delivery, 213–220 controlled drug delivery, nanoporous implants for,
263–283
biocompatibility of nanoporous implants, 277–283 long-term lysozyme diffusion studies, -279–281 post-implant diffusion data, 282–283
in vivo biocompatibility evaluation, 278–279 in vivo/in vitro correlation, 281–282
conclusions, 283
fabrication of nanoporous membranes, 269–271 implant assembly and loading, 271 introduction, 263–269
comparison of nanopore technology with existing drug delivery technologies, 267–269
concept of controlled drug delivery, 263–264 nanopore technology, 264–276
cross-sectional view of nanochannel delivery system, 264–276
nanoporous implant diffusion studies, 271–277 bovine serum albumin release data, 273–275 interferon release data, 272–273
modeling and data fitting, 276–277 results interpretation, 275–276
controlled drug release, 213–220 common “soft” materials used in, 215
future of nanotechnology in pharmaceutical research, 219
“obedient” materials, 216
photonic crystals as self-reporting biomaterials, 217
porous material-based systems, 214–215 porous silicon, 216–217
silicon-based porous materials, 215–216 system design considerations, 214 templated nano materials, 217
template for optical nanostructures, 217–219 CT (computerized tomography), 107, 200, 204 cyclosporin, 20197
cystic fibrois, 194
cytoadhesive oral drug delivery, 237
DBS (deep brain stimulation), 104, 105 DEP (dielectrophoresis), 338
diabetes, 172, 187, 214, 318, 334
diffusion immunoassay, T-sensor device for, 72 DNAase, 197–198
drug delivery. See also controlled drug delivery, nanoporous implants for; transdermal drug delivery, using low-frequency sonophoresis
localizing using nanoparticles, 204