
Therapeutic Micro-Nano Technology BioMEMs - Tejlal Desai & Sangeeta Bhatia
.pdfMEMS AND NEUROSURGERY |
121 |
[2]E.C. Benzel. Biomechanics of Spine Stabilization. Rolling Meadows, IL, American Association of Neurological Surgeons, 2001.
[3]P.M. Black. Harvey cushing at the Peter Brigham hospital. Neurosurgery, 45:990–1001, 1999.
[4]D. Campillo. Neurosurgical pathology in prehistory. Acta Neurochirurg., 70:275–290, 1984.
[5]J.M. Drake, J.R.W. Kestle, and S. Tuli. CSF shunts 50 years on—past, present and future. Child’s Ner. Syst., 16:800–804, 2000.
[6]J.M. Drake and C. Sainte-Rose. The Shunt Book. Cambridge, MA, Blackwell Science, 1995.
[7]R. Drucker-Colin and L. Verdugo-Diaz. Cell transplantation for Parkinson’s disease: present status. Cell. Mol. Neurobiol., 24:301–316, 2004.
[8]S. El Gindi. Neurosurgery in Egypt: past, present, and future—from pyramids to radiosurgery. Neurosurgery, 51:795–796, 2002.
[9]S.T. Goodrich. History of spine surgery in the ancient and medieval worlds. Neurosurg. Focus, 16:E2, 2004.
[10]S.H. Greenblatt. The crucial decade: modem neurosurgery’s definitive development in Harvey Cushing’s early research and practice, 1900 to 1910. J. Neurosurg., 87:964–971, 1997.
[11]D.R. Groothuis. The blood-brain and blood-tumor barriers: a review of strategies for increasing drug delivery.
Neuro-Oncology, 2:45–59, 2000.
[12]C. Guerin, A. Olivi, J.D. Weingart, H.C. Lawson, and H. Brem. Recent advances in brain tumor therapy: local intracerebral drug delivery by polymers. Investigat. New Drugs, 22:27–37, 2004.
[13]R.D. Guyer and D.D. Ohnmeiss. Intervertebral disc prostheses. Spine, 28:S15–S23, 2003.
[14]L.F. Haas. Papyrus of Ebers and Smith. J. Neurol., Neurosurg., Psychiat., 67:578, 1999.
[15]B. Karger, S. Hubert, and B. Brinkmann. Arrow wounds: major stimulus in the history of surgery. World J. Surg., 25:1550–1555, 200l.
[16]A.H. Kaye and E.R. Laws, Jr. Churchill Livingstone, London, New York, 2001.
[17]W. Kohen, C. Kolbenschlag, S. Teske-Keiser, and B. Jansen. Development of a long-lasting ventricular catheter impregnated with a combination of antibiotics. Biomaterials, 24:4865–4869, 2003.
[18]J.G. Lascaratos, I.G. Panourias, and D.E. Sakas. Hydrocephalus according to Byzantine writers. Neurosurgery, 55:214–220, 2004.
[19]E.R. Laws, Jr. Neurosurgery’s man of the century: Harvey Cushing—the man and his legacy. Neurosurgery, 45:977–982, 1999.
[20]O. Lindvall, Z. Kokaia, and A. Martinez-Serrano. Stem cell therapy for human neurodegenerative disordershow to make it work. Nat. Med., 10:S42–S50, 2004.
[21]C.Y. Liu and M.L.J. Apuzzo. The genesis of neurosurgery and the evolution of the neurosurgical operative environment: part I-prehistory to 2003. Neurosurgery, 52:3–19, 2003.
[22]C.Y. Liu, M. Spicer, and M.L.J. Apuzzo. The genesis of neurosurgery and the evolution of the neurosurgical operative environment: part II-concepts for future development, 2003 and beyond. Neurosurgery, 52:20–35, 2003.
[23]G. Martin. Was Hippocrates a beginner at trepanning and where did he learn? J Clin. Neurosci., 7:500–502, 2000.
[24]D.E. McDonnell. History of spinal surgery: one neurosurgeon’s perspective. Neurosurg. Focus, 16:El, 2004.
[25]E.B. Montgomery. Two advancements in the management of Parkinson disease. Cleveland Clin. J. Med., 69:639–643, 2002.
[26]R.A. Morantz and J.W. Walsh. Marcel Dekker, New York, 1994.
[27]J. Piek, G. Lidke, T. Terberger, U. von Smekal, and M.R. Gaab. Stone age surgery in MeckelburgVorpommem: a systematic study. Neurosurgery, 45:147–151, 1999.
[28]I.K. Pople. Hydrocephalus and shunts: what the neurologist should know. J. Neurol. Neurosurg. Psych., 73:i17–i22, 2002.
[29]D. Purves, G.J. Augustine, D. Fitzpatrick, L.C. Katz, A.-S. LaMantia, J.O. McNamara, and S.M. Williams. Sinauer Associates, Sunderland, MA, 2001.
[30]G.E. Rawlings, 3rd, and E. Rossitch, Jr. The history of trephination in Africa with a discussion of its current status and continuing practice. Surg. Neurol., 41:507–513, 1994.
[31]S.S. Rengachary and R.H. Wilkins. Mosby, St. Louis, 1994.
[32]M. Rutkow. Trephination: how did they do it? Archives of Surgery, 135:1119, 2000.
[33]J.L. Stone Paul Broca and the first craniotomy based on cerebral localization. J. Neurosurg. 75:154–159, 1991.
[34]C. Toporek and K. Robinson. Hydrocephalus: A Guide for Patients, Families, and Friends. Beijing; Sebastopol, O’Reilly, 1999.
122 |
SHUVO ROY ET AL. |
[35]M. Victor and A.H. Ropper McGraw-Hill, New York, 2001.
[36]L.W. Way and G.M. Doherty. McGraw-Hill, New York, 2003.
[37]T.A. Zesiewicz and R.A. Hauser Neurosurgery for Parkinson’s disease. Seminar. Neurol., 21:91–101, 200l.
[38]E. Benzel, L. Ferrara, S. Roy, and A. Fleischman. Micromachines in spine surgery. Spine, 29:601–606, 2004.
[39]E.C. Benzel. Biomechanics of Spine Stabilization. Rolling Meadows, IL, AANS Publications, 2001.
[40]E.C.Benzel, L.A. Ferrara, S. Roy, and A.J. Fleischman. Biomaterials and implantable devices: discoveries in the spine surgery arena. Clini. Neurosurg., 49:209–225, 2002.
[41]S. Chung, J.K. Kim, K.C. Wang, D.-C. Han, and J.-K. Chang. Development of MEMS-based cerebrospinal fluid shunt system. Biomed. Microdev., 5:311–321, 2003.
[42]T.A. Desai, W.H. Chu, J.K. Tu, G.M. Beattie, A. Hayek, and M. Ferrari. Microfabricated immunoisolating biocapsules.Biotechno. Bioeng., 57:118–120, 1998.
[43]D.J. Edell. A peripheral nerve information transducer for amputees: long-term multichannel recordings from rabbit peripheral nerves. IEEE Trans. Biomed. Eng., 33:203–214, 1986.
[44]D.J. Edell, V.V. Toi, V.M. McNeil, and L.D. Clark. Factors influencing the biocompatibility of insertable silicon microshafts in cerebral cortex. IEEE Trans. Biomed. Eng., 39:635–643, 1992.
[45]L.A. Ferrara, A.J. Fleischman, E.C. Benzel, and S. Roy. Silicon dermabrasion tools for skin resurfacing applications. Med. Eng. Phys., 25:483–490, 2003.
[46]L.A. Ferrara, A.J. Fleischman, D. Togawa, T.W. Bauer, E.C. Benzel, and S. Roy. An in vivo biocompatibility assessment of MEMS materials for spinal fusion monitoring. Biomed. Microdev., 5:297–302, 2003.
[47]A.J. Fleischman. Miniature high frequency focused ultrasonic transducers for minimally invasive imaging procedures. Sens. Actu. A: Phys., 103:76–82, 2003.
[48]W.M. Grill, J.W. McDonald, P.H. Peckham, W. Heetderks, J. Kocsis, and M. Weinrich. At the interface: convergence of neural regeneration and neural prostheses for restoration of function. J. Rehabilitat. Res. Develop., 38:633–639, 2001.
[49]J.W. Judy. International Conference of IEEE Engineering in Medicine and Biology Society. Cancun, MEXICO, 2003.
[50]D.T. Kewley, M.D. Hills, D.A. Borkholder, I.E. Opris, N.I. Maluf, C.W. Storment, J.M. Bower, and G.T.A. Kovacs. Plasmaetched neural probes. Sen. Actu., A58:27–35, 1997.
[51]W.H. Ko, C.W. Meyrick, and H.L Rekate. Cerebrospinal fluid control system. Proceedings of the IEEE, 76: 1226–1235, 1988.
[52]G. Kotzar, M. Freas, P. Abel, A. Fleischman, S. Roy, C. Zorman, J.M. Moran, and J. Melzak. Evaluation of MEMS materials of construction for implantable medical devices. Biomaterials, 23:2737, 2002.
[53]G.T.A. Kovacs, C.W. Storment, and J.M. Rosen. Regeneration microelectrode array for peripheral nerve recording and stimulation. IEEE Trans. Biomed. Eng., 39:893–902, 1992.
[54]J.S. Kroin, R.J. McCarthy, L. Stylos, K. Miesel, A.D. Ivankovich, and R.D. Penn. Long-term testing of an intracranial pressure monitoring device. J. Neurosurg., 93:852–858, 2000.
[55]K.S. Lebouitz and M. Migliuolo. Verimetra, Inc., USA, 2002.
[56]E.H. Ledet, B.L. Sachs, J.B. Brunski, C.E. Gatto, and P.S. Donzelli. Real-time in vivo loading in the lumbar spine: part 1. Interbody implant: load cell design and preliminary results. Spine, 25:2595–2600, 2000.
[57]K.-K. Lee, J. He, A. Singh, S. Massia, G. Ehteshami, B. Kim, and G. Raupp. Polyimide-based intracortical neural implant with improved structural stiffness. J. Micromech. Microeng., 14:32–37, 2004.
[58]A.M. Leung, W.H. Ko, T.M. Spear, and J.A. Bettice. Intracranial pressure telemetry system using semicustom integrated circuits. IEEE Trans. Biomed. Eng., 33:386–395, 1986.
[59]L.-A. Liew and V.M. Bright. 2000 IEEE-EMBS International Special Topic Conference on Microtechnologies in Medicine and Biology, Lyon, FRANCE, 2000.
[60]C.Y. Liu, M. Spicer, and M.L.J. Apuzzo. The genesis of neurosurgery and the evolution of the neurosurgical operative environment: part II-concepts for future development, 2003 and beyond. Neurosurgery, 52:20–35, 2003.
[61]M.P. Maher, J. Pine, J. Wright, and Y.-C. Tai. The neurochip: a newmultielectrode device for stimulating and recording from cultured neurons. J. Neurosci. Methods, 87:45–56, 1999.
[62]D.V. McAllister, M.G. Allen, and M.R. Prausnitz. Microfabricated microneedles for gene and drug delivery.
Ann. Rev. Biomed. Eng., 2:289–313, 2000.
[63]A.F. Mensinger, D.J. Anderson, C.J. Buchko, M.A. Johnson, D.C. Martin, P.A. Tresco, R.B. Silver, and S.M. Highstein. Chronic recording of regenerating VIIIth nerve axons with a sieve electrode. J. Neurophys., 83:611–615, 2000.
MEMS AND NEUROSURGERY |
123 |
[64]P.S. Motta and J.W. Judy. In IEEE-EMBS Special Topic Conference on Microtechnologies in Medicine and Biology. Madision, WI, pp. 251–254, 2002.
[65]P.S. Motta and J.W. Judy. Solid-State Sensor and Actuator Workshop. Hilton Head, SC, 2002.
[66]K. Najafi. Solid-state microsensors for cortical nerve recordings. IEEE Eng. Med. Biol., 13:375–387, 1994.
[67]K. Najafi and J. Hetke. Strength characterization of silicon microprobes in neurophysiological tissues. IEEE Trans. Biomed. Eng., 37:474–481, 1990.
[68]K. Najafi, K.D. Wise, and T. Mochizuki. A high-yield IC-compatible multichannel recording array. IEEE Trans. Elect. Dev., 32:1206–1211, 1985.
[69]D.L. Polla, A.G. Erdman, W.P. Robbins, D.T. Markus, J. Diaz-Diaz, R. Rizq, Y. Nam, H.T. Brickner, A. Wang, and P. Krulevitch. Microdevices in medicine. Annu. Rev. Biomed. Eng., 2:551–576, 2000.
[70]P.J. Rousche, D.S. Pellin, D.P. Pivin, J.C. Williams, R.J. Vetter, and D.R. Kipke. Flexible polyimide based intracortical electrode arrays with bioactive capability. IEEE Trans. Biomed. Eng., 48, 2001.
[71]S. Roy, L.A. Ferrara, A.J. Fleischman, and E.C. Benzel. Microelectromechanical systems and neurosurgery: a new era in a new millennium. Neurosurgery, 49:779–797; (discussion) 797–778, 2001.
[72]S. Roy and A.J. Fleischman. Cytotoxicity evaluation for microsystems materials using human cells. Sens. and Mat., 15:335–340, 2003.
[73]S. Roy and M. Mehregany., Introduction to MEMS. In H. Helvajian (ed.), Microengineering Aerospace Systems, The Aerospace Press, El Segundo, CA, pp. 1–28, 1999.
[74]B. Schlosshauer, T. Brinker, H.-W. Muller, J.-U. Meyer. Towards micro electrode implants: in vitro guidance of rat spinal cord neurites through polyimide sieves by Schwann cells. Brain Res., 903:237–241, 2001.
[75]A. Singh, K. Lee, J. He, G. Ehteshami, S. Massia, and G. Raupp. International Conference of IEEE Engineering in Medicine and Biology Society Cancun, MEXICO, pp. 3364–3367, 2003.
II
Drug Delivery
7
Vascular Zip Codes and
Nanoparticle Targeting
Erkki Ruoslahti
The Burnham Institute, Cancer Research Center, 10901 North Torrey Pines Road, La Jolla, CA 92037
The use of nanoparticles in medicine will require sensors that can serve as guidance molecules for targeting the particles to appropriate sites in the body. Another set of sensors will be needed to allow the particles to respond to features at the target, such as inflammation, cell death, etc. Compounds that target the vascular endothelium provide one answer to the guidance and sensing problems. The endothelium of blood vessels is readily accessible from the blood stream, and the vessels in different tissues carry unique molecular signatures. Pathological lesions also put their signature on the vasculature; in tumors, both blood and lymphatic vessels differ from normal vessels. Peptides and antibodies that recognize vascular signatures have been shown to be useful in directing therapeutic agents to targets such as tumors. The targeting can enhance the efficacy of the therapy while reducing side effects. Combining the targeting technology with nanoparticles can take us a step closer to truly smart nanodevices.
7.1. INTRODUCTION
The concept of a “magic bullet” as a targeted treatment of disease has been around since Paul Ehrlich realized the unique specificity of antibodies. There are two ways of designing a targeted therapy: a drug or other therapeutic moiety can be so specific that it will affect only the intended target, or it can be physically targeted to the appropriate site in the body. Physical targeting could potentially make it possible to design “smart” nanoparticles that seek out the site of disease. The targeting would concentrate the particles at that site for diagnostic or therapeutic purposes. Targeted nanoparticles have been designed and some of
128 |
ERKKI RUOSLAHTI |
them are about to enter clinical trials [31, 41]. The target recognition event could also serve as a sensor that would trigger a change in the particle. This change could then be detectable for diagnostic purposes, or could trigger the release of a drug or other treatments. The result would be a smart nanodevice of a kind that does not exist yet.
Vascular addresses can serve in targeting of diagnostic and therapeutic agents, including nanoparticles and nanodevices. The vasculature is an attractive tissue for targeting purposes because it is accessible through the blood stream and because extensive heterogeneity exists in endothelial cells that form the inner lining of blood vessels and lymphatics. Individual tissues express their own endothelial markers, and the vessels in pathological lesions, such as tumors, differ from the vessels in normal tissues [37]. In this chapter, I review some of the recent developments in vascular addresses and discuss the use of probes that recognize such addresses in nanoparticle targeting.
7.2. IN VIVO PHAGE DISPLAY IN VASCULAR ANALYSIS
We use bacteriophage, a virus that infects common bacteria, as a tool in probing the vasculature for specific changes. The phage can be engineered to display a vast collection (library) of peptides (more than 109 of them) on its surface. By injecting such a library into the blood stream of a live mouse, we can select peptides that make the phage home to a given target [4, 7, 22, 33]. We have also recently expanded the tissue targeting to include the screening of libraries of small molecular weight chemicals [7]. When screening phage libraries, we intravenously inject the library into a mouse, rescue the phage from the target tissue, and repeat the process several times to derive a phage pool that selectively homes to the target tissue. More recently, we have included an ex vivo step to generate a phage pool enriched in sequences capable of recognizing the target tissue [22]. The homing peptides and their binding molecules (receptors) discovered in this manner have revealed a wealth of heterogeneity in the blood and lymphatic vessels (Fig. 7.1). These results are discussed next.
7.3. TISSUE-SPECIFIC ZIP CODES IN BLOOD VESSELS
Our phage studies, as well as phage and antibody studies by others, have revealed an unexpected extent of tissue-specific molecular heterogeneity in the vasculature of various normal tissues. Tissue-specific vascular homing peptides have been obtained for at least 15 different tissues [3, 13, 17, 32, 39]. Importantly, we have been successful with each individual normal tissue we have chosen for targeting so far, suggesting that every tissue puts its own signature on the vasculature. The list includes both major organs, such as the brain, lungs, heart, and kidneys, and minor ones such as the prostate [3, 17, 39]. With our current technology, we obtain phage that home to the target tissue with a selectivity that can reach several hundred fold [22, 42]. Only a few of the molecules that the tissue-specific vascular homing peptides or antibodies recognize are known. Proteases are strongly represented among them: dipeptidyl peptidase IV [25] and membrane dipeptidase [36] are markers of lung vasculature; and another peptidase, aminopeptidase P, is selectively expressed in breast gland vasculature [17]. However, other types of molecules can also serve as tissue-specific endothelial markers [42].
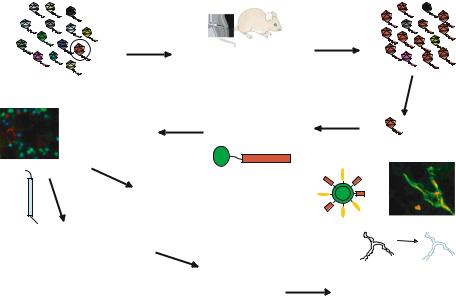
VASCULAR ZIP CODES AND NANOPARTICLE TARGETING |
129 |
Inject phage library into |
Isolate phage from |
a mouse’s tail vein |
target tissue |
Specific phage binds to blood vessels in target tissue
Inject peptide to study |
Synthesize FITC- |
|
|
labeled peptide |
|
tissue localization |
|
|
|
|
|
|
F |
PEPTIDE |
|
I |
|
|
|
Use the peptide to direct drugs, genes, nanoparticles, etc into target issues
Identify receptor for the peptide by affinity chromatography or cDNA cloning
Use the receptor to prepare antibodies and other ligands
Sequence insert in enriched phage
Test the antibodies, ligands, siRNA, etc to determine receptor function and potential as drug target
FIGURE 7.1. Schematic representation of the protocol for in vivo phage screening and homing peptide characterization. A library containing as many as several billion peptides and expressed on the surface of bacteriophage is intravenously injected into a mouse. A few minutes later, the target tissue is removed, and a phage pool enriched in phage carrying homing peptides for the target tissue is rescued from it by infecting bacteria. A highly enriched pool is obtained by repeating the process with the first pool. Sequencing of the DNA encoding the peptides displayed by the phage reveals the sequences of the enriched peptides. The peptides can be used to deliver labeling molecules (micrograph on the left; [35]), drugs, or nanoparticles to a target tissue. A quantum dot coated with a peptide (for homing) and polyethylene glycol (for solubility) is schematically depicted [2]. The micrograph on the right shows green tumor-homing quantum dots that have accumulated in tumor vessels (labeled with a red dye). The homing peptides can also be used to identify the receptors for the peptides. Some of the receptors are functionally important in the target vasculature, and compounds that affect their function may destroy the target vasculature [29].
7.4. SPECIAL FEATURES OF VESSELS IN DISEASE
It is well known that inflammation causes changes in the endothelium of adjacent vessels. The expression of adhesion molecules that bind leukocytes from blood is increased, and the production of cytokines that attract and activate leukocytes is turned on (Springer, 1994). Among other pathologies, the molecular features that characterize the vasculature of regenerating tissues and tumors have been extensively studied. In both cases, new vessels sprout from existing ones in a process termed angiogenesis, and the growing vessels are different from normal vessels. Further stimulating the growth of angiogenic vessels, or targeting them for destruction are important therapeutic goals; the former to facilitate regeneration of damaged tissue, and the latter to starve a tumor.
The markers of angiogenesis include increased expression of receptors for vascular endothelial growth factor (VEGF) receptors [1] and integrins [15, 27]. Indeed, one of the peptides identified by in vivo screening of phage libraries for tumor homing recognizes
130 |
ERKKI RUOSLAHTI |
αvβ3 and αvβ5 [4], two of the integrins that are selectively expressed in angiogenic vessels. Other proteins selectively expressed in angiogenic vessels include aminopeptidase N; its involvement in angiogenesis was established by in vivo phage display [34]. The form of aminopeptidase N expressed in tumor vasculature may be distinct from those expressed by normal cells [10]. A similar situation exists with a fibronectin splicing variant that is selectively expressed in angiogenic blood vessels, whereas fibronectin, in general, is ubiquitous [20].
We recently used one of our tumor-homing peptides to show that angiogenic endothelial cells, unlike resting endothelial cells, express nucleolin at the cell surface [8]. Antibodies prepared against nucleolin also specifically recognize blood vessels both in tumors and in a model of wound healing angiogenesis. Various tumor cells also express cell surface nucleolin. Thus, peptides, antibodies, and nucleic acid probes that bind to nucleolin can specifically target both tumor blood vessels and tumor cells. Nucleolin can serve as a receptor for tumor-targeting quantum dots [2].
Detailed analysis of the specificity of the tumor-homing peptides we have selected from phage libraries shows that some of the peptides recognize vascular markers that are common to all tumors, but some only bind to the vessels in a limited range of tumors. The former recognize angiogenesis-related proteins. The molecules recognized by the peptides reactive with a restricted range of tumors are not known, but it is likely that the tumor tissue sends the endothelium the signals necessary for the expression of specific molecules. We are currently attempting to identify tumor-homing peptides that would be specific for the blood vessels or lymphatics of a given tumor type, such as prostate cancer or breast cancer.
We have recently discovered an additional degree of vascular diversity in tumors. A collaborative study with Dr. Douglas Hanahan’s laboratory using transgenic mouse tumor models showed that some homing peptides were capable of distinguishing between the vessels of pre-malignant and malignant lesions (while not recognizing the normal vasculature). This finding indicates that the molecular features evolve as tumorigenesis progresses [22, 26]. Interestingly, the islet tumor set of peptides did not recognize the various stages of the skin tumors, and vice versa. These studies reveal an unexpected degree of diversity in the vasculature of tumors and premalignant lesions. As in vivo phage display and similar analyses are extended beyond tumor vasculature, it is likely that vascular markers for lesions in other diseases will be identified. Initial studies on atherosclerotic plaques have already shown promise in this regard [24].
The angiogenesis-specific proteins revealed by phage display are likely to be functionally important in the angiogenesis process. One of the first tumor-homing peptides we found was a peptide containing the RGD (arginine-glycine-aspartic acid) sequence, which we had many years ago shown to be central to the recognition of extracellular matrix proteins by integrins [38]. This particular peptide homes to tumor vasculature because it is specific for the αvβ3 and αvβ5 integrins, which are up-regulated in angiogenic vessels [37]. Blocking the activity of these integrins is known to inhibit angiogenesis [16]. Aminopeptidase N, identified as an angiogenesis marker with another phage peptide, is also functionally important in angiogenesis [34]. A third example is provided by cell surface-expressed nucleolin. Anti-nucleolin antibodies (S. Christian, M. Akerman, J. Pilch, and E. Ruoslahti, in preparation) inhibit angiogenesis, and nucleolin-binding aptamers suppress tumor cell proliferation [5]. Finally, a peptide that recognizes tumor endothelial cells in tumor lymphatics
VASCULAR ZIP CODES AND NANOPARTICLE TARGETING |
131 |
and also binds to tumor cells [28] inhibits tumor growth when systemically administered to tumor-bearing mice [29]. These results underscore the point that the molecular markers of tumor vasculature are also likely to be functionally important for the maintenance of the vasculature. Moreover, the lymphatic homing peptide provided the first demonstration that tumor lymphatics are molecularly different from normal lymphatics. The availability of peptides that home to tumor lymphatics, along with peptides that home to tumor blood vessels, may allow a two-pronged attack on tumors.
7.5. DELIVERY OF DIAGNOSTIC AND THERAPEUTIC AGENTS TO VASCULAR TARGETS
Peptides that home to specific vascular sites, such a tumors, can carry a payload to that site. When the payload is a drug, the delivery will concentrate the drug at the target site, and increased efficacy and lesser side effects should result. We have used the peptides that specifically bind to the αvβ3 and αvβ5 integrins (RGD motif peptide) or to aminopeptidase N (NGR motif peptide) to target doxorubicin in this manner [4]. Another laboratory has used these same peptides to deliver tumor necrosis factor α into tumors [9, 10].
A particularly illuminating example of homing peptide targeting is our use of the RGD and NGR peptides to target an anti-bacterial peptide to the vasculature at various disease sites. The payload in this case is an amphipathic peptide that disrupts bacterial and mitochondrial membranes and induces apoptosis if internalized by mammalian cells. Systemic treatment of tumor-bearing mice with conjugates in which this pro-apoptotic peptide is coupled to one of the homing peptides inhibited tumor growth [14]. Similar treatment also suppressed inflammation in arthritic synovium, which exhibits strong angiogenesis [19]. Moreover, combining the same pro-apoptotic peptide with a homing peptide that binds to the blood vessels of the normal prostate yielded a compound that caused partial destruction of the prostate tissue and delayed the development of prostate cancer in mice transgenic for a prostate-expressed oncogene [3]. These results, better than anything, demonstrate the power of the homing peptide targeting; the same essentially non-specific toxic peptide affects different tissues, depending on its homing peptide partner.
7.6. HOMING PEPTIDES FOR SUBCELLULAR TARGETING
Some of our homing peptides are capable of being internalized by the target cells and delivering a drug-like payload (fluorescein, rhodamine, or biotin), into the cell nucleus (Fig. 7.2; [22, 26, 28, 35]). These internalizing peptides contain numerous basic amino acid residues, which apparently are important for the internalization and may form a nuclear localization signal. Alternatively, the peptide may be binding to a transport molecule that shuttles into the nucleus. This appears to be the case with a peptide that binds to cell surface nucleolin and is internalized and transported into the nucleus [8]. These internalizing peptides may prove to be particularly useful for delivering therapeutic agents that act in the nucleus. Bhatia and coworkers have done work on quantum dots targeted to various subcellular organs [12]. Subcellular targeting, along with tissue targeting, is likely to become an important aspect of cellular level nanomedicine.

132 ERKKI RUOSLAHTI
F3 tumor-homing |
|
peptide |
Tat peptide |
|
|
F3 + anti- |
F3 |
nucleolin |
|
Tat + anti- |
Tat |
nucleolin |
FIGURE 7.2. Cell penetrating homing peptides. F3 is an example of recently isolated homing peptides that are internalized by their target cells. F3 binds to angiogenic endothelial cells and certain tumor cells [8, 35]. Shown is the internalization of fluorescein-labeled F3 by cultured tumor cells (upper left panel). The F3 peptide resembles cell penetrating peptides such as the Tat peptide [30] in its ability to carry a payload to a cell, with the important difference that F3 is cell type-specifc and has a distinct receptor, cell surafe-expressed nucleolin. Anti-nucleolin inhibits the uptake of F3 (upper right panel), but has no effect on the intenalization of fluorescein-labeled Tat peptide (lower panels). Modified from [8], with permission.
7.7. NANOPARTICLE TARGETING
Vascular homing peptides can deliver particles to a specific tissue site. The phage we use to probe the vascular heterogeneity is a nanoparticle. The phage particle actually is a sophisticated nanodevice, as it functions like a nanoscale syringe that injects the phage’s genome into host bacteria. Animal viruses used as gene therapy vectors have also been