
Therapeutic Micro-Nano Technology BioMEMs - Tejlal Desai & Sangeeta Bhatia
.pdf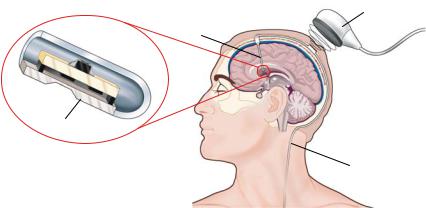
MEMS AND NEUROSURGERY |
|
111 |
|
Ventricular |
Wireless |
|
Telemetry |
|
|
Catheter |
|
|
Module |
|
|
|
Pressure Sensor
Distal
Catheter
FIGURE 6.11. Schematic depiction of a concept for an intracranial pressure (ICP) measurement system. A MEMS pressure sensor is located at the tip of the ventricular catheter. The pressure information is transmitted via wireless telemetry from an implanted coil to an external telemetry module.
subacute and long term basis) would be substantial. Decisions regarding treatment could be readily made and catastrophic neurologic deterioration could be prevented or aborted.
Figure 6.11 presents a schematic overview of a proposed Programmable Automatic Shunt System for the treatment of hydrocephalus [51]. The design of the shunt system incorporates, in a single implanted unit, MEMS sensors for the measurement of ICP and cerebrospinal fluid (CSF) flow, an adjustable valve for CSF drainage, and microelectronics for signal processing and telemetry [58]. The implant transmits the pressure and flow information via the implanted coil antenna to the neurosurgeon, who, in turn, issues commands to adjust the CSF drainage valve. The system could potentially be modified to work based on internal feedback without neurosurgeon input. This latter strategy embraces and, in fact defines, the concept of a smart system—or in the specific case discussed here, a “smart shunt.” With such a smart shunt, for example, an elevation of intracranial pressure would signal a modification of outflow resistance so that CSF flow would be increased. Recent efforts in the development of a smart shunt system include the investigations into microvalves and packaging strategies [41, 59].
The employment of intracranial pressure monitoring techniques may even be applied outside the immediate medical environment (e.g., hospital or clinic). For example, the employment of MEMS-derived pressure sensing technology with modern telemetric capabilities might permit a parent of a child with hydrocephalus to monitor intracranial pressure. Such a system could alert the parent to an impending catastrophe related to increased cerebrospinal fluid pressure, well in advance of clinical manifestations. A system in which a “red light—yellow light—green light” concept is employed could be used to alert a parent via an external sensing device that is simply positioned close to the child’s head with telemetric and MEMS technology. Then, an evaluation of the child’s intracranial pressure could be made and grossly presented to the parent in the form of a “green light, yellow light, or red light”, thus providing an early warning system. The neurosurgeon could then act accordingly following a call from the parents. Finally, the surgeon should have, in his/her office, a monitoring unit that would provide precise pressure data and
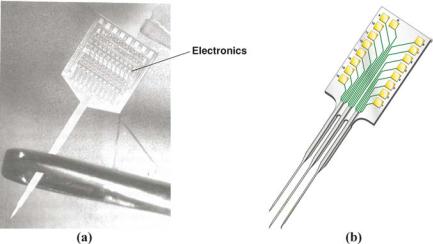
112 |
SHUVO ROY ET AL. |
waveforms. This approach would facilitate further analysis of the patient’s status, thus making the clinical decision-making process more accurate and safer.
6.7.2. Neural Prostheses
Neural prostheses include devices that facilitate the manipulation of the nervous system. They may be used to augment or diminish (e.g., ablate) neurological function. They are becoming increasingly important in neurosurgical applications due to the myriad of recent advances in the functional neurosurgery and surgical epilepsy arenas. With such devices as implanted electrodes for the modulation of undesirable spontaneous motion in Parkinson’s patient, feedback to the surgeon and to the device itself (smart system) may be of extreme clinical significance. For example, rather than providing a fixed electrical current pattern to a specific region of the brain to compensate for a pathological movement, a “smart prosthesis” could record electrical activity from the surrounding brain and modify electrode stimulation, based on the input from the surrounding brain. This type of input could perhaps even help the surgeon direct the initial placement of the electrode. Furthermore, integration of locomotive actuators onto the electrodes could enable the creation of a “smart neural prosthesis” that would propel itself to a more optimal recording and stimulation location.
Figure 6.12 presents examples of microelectrode arrays that have been fabricated using MEMS technology to link the nervous system to microelectronics circuitry [50, 66, 68]. These neural probes were designed for multichannel sensing and stimulation in the cerebral cortex. The micromachined silicon substrate supports an array of metallic film conductors that are insulated above and below by dielectric films. Openings in the upper layer along the probe shank defined stimulating or recording sites, which are inlayed with gold or iridium oxide for interfacing with brain tissue. At the rear of the probe, integrated circuits provided
FIGURE 6.12. Examples of neural microprobes fabricated by MEMS technology. (a) Photograph showing integrated electronics and microprobe shank, which is passed through the eye of a needle. (b) Schematic depiction of a multi-shank probe.
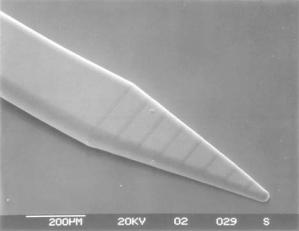
MEMS AND NEUROSURGERY |
113 |
FIGURE 6.13. SEM image of an electroplated microprobe for deep brain stimulation (DBS) applications. This probe design exhibits smooth geometrical taper while maintaining sharp tips necessary for ready penetration into brain tissue. (Courtesy: UCLA)
signal amplification, filtering, and a multiplexed interface to the output leads, which are connected to external circuitry through a percutaneous plug. The applicability of this neural prosthesis system could be further enhanced if the electrical connection through the wires was substituted by wireless telemetry.
Recent investigations into the use of MEMS technology for the development of neural probes have focused on addressing biocompatibility for long-term performance, integration of fluidic ports for drug delivery, and novel fabrication strategies to achieve shank flexibility and three-dimensional geometry [49, 64]. Flexibility is desirable to minimize tissue damage that can be caused by relative micro-motion between the implanted shank and the brain. However, the shank must be sufficiently stiff to penetrate into the brain without buckling. One feasible strategy to meet the structural criteria appears to be a combination shank comprising a polymeric material, such as polyimide or benzocyclobutene (BCB), and silicon [57, 75]. Other considerations for penetration of neural probes into the brain are requirements for sharp tips and smooth geometrical taper. A MEMS probe that meets these criteria is shown in Figure 6.13 [65]. The probe design could incorporate multiple electrodes while promising to maintain the taper and length characteristics of the conventional micro-wires.
6.7.3. Drug Delivery Systems
Drugs and other substances, that require precise instillation techniques, could be delivered by MEMS systems. This is particularly so for agents that require micro dosages (e.g., bone morphogenic proteins, some chemotherapeutic agents, or dopamine and related agents). Such a device could be placed at the time of a surgical fusion procedure to stimulate bone fusion, within a tumor bed to precisely apply chemotherapeutic agents, or into the deep brain substance to treat movement disorders. A controlled and precise drug release could then be achieved.
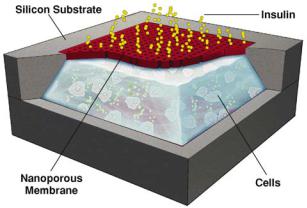
114 |
SHUVO ROY ET AL. |
FIGURE 6.14. Schematic cut-away depiction of a MEMS biocapsule for transplantation of pancreatic islet cells. The nanometer-sized pores in the membrane allow for nutrient and insulin transport but ensure an immunoprivileged environment for the islet cells.
Figure 6.14 presents a schematic depiction of a micromachined silicon immunoisolating biocapsule that was developed to house pancreatic islet cell transplants for insulin therapy [42]. This MEMS device consists of a cell chamber and filter membrane with 20 nm pores. The pore size is large enough to allow for insulin and oxygen exchange, but small enough to impede the passage of viruses and immune molecules. The microfabricated biocapsule could also be used in the neurosurgical arena to place neuronal xenografts into the brain. The transplanted neurons could be used to supplement electrical stimulation or lesion surgery for neurologically impaired patients. The biocapsule could also be used to create drug delivery systems in which the release of the drug is controlled by its diffusion across the micromachined membrane, while the membrane protects the patient and the xenograft by creating an immunoprivileged environment within the capsules.
6.7.4. Smart Surgical Instruments and Minimally Invasive Surgery
The capability of MEMS could enable a set of next-generation surgical tools. For example, the incorporation of pressure sensors, strain gauges, or biochemical sensors into surgical instruments could enable smart instruments that would be able to distinguish between different types of tissue, both with regard to tissue density and biochemical makeup. Furthermore, the incorporation of MEMS sensors and actuators could allow for precision cutting or local manipulation of tissue with unprecedented control, thereby minimizing nondesirable tissue damage. A possible example of such smart tools is illustrated in Figure 6.15 [55]. The employment of sensors and actuators that modify, limit, and amplify selected surgeon-generated motion could significantly enhance surgeon precision, accuracy, and speed. This can be accomplished by the employment of systems that, among other things, filter out non-desirable repetitive motion (e.g., tremors), while enhancing desirable motion (e.g., precision) and even amplifying other motions (e.g., extension of an instrument, such as
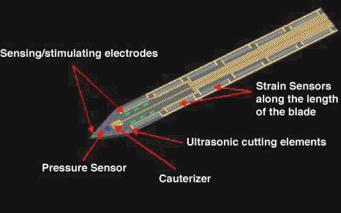
MEMS AND NEUROSURGERY |
115 |
FIGURE 6.15. Schematic illustration of a smart tool for minimally invasive surgery. The sensors would enable the neurosurgeon to distinguish between different types of tissue, while the ultrasonic cutting elements and cauterizer would enable precision cuts with minimal non-specific damage. (Courtesy: Verimetra Inc)
a micro-dissection tool, under enhanced control). The availability of such smart instruments should also enhance the capabilities of minimally invasive procedures in neurosurgery.
The use of piercing probes and cutting blades for neurosurgical applications ranges from needles for micro-syringes to scalpels for surgery. These pointed and edged tools are typically mass-produced with metals (e.g., stainless steel) and glass, or are individually hand-ground from harder materials, such as diamond and ceramics. The mass-produced components may be inexpensive. However, they may become blunt and degrade relatively quickly over time. In contrast, diamond and ceramic-based tools exhibit better performance, but at relatively higher cost. The use of MEMS technology for the fabrication of silicon probes and blades combine the performance characteristics of the hard-material tools with the cost advantages of mass production. Specifically, the miniaturization and batch fabrication characteristics of MEMS technology enable the fabrication of arrays of microprobes and microblades simultaneously on a single substrate. In contrast to the grinding processes used to sharpen metallic tools, the etching processes used to create microfabricated devices could provide smoother surfaces and sharper edges, as shown in Figure 6.16 [45]. Consequently, meticulous dissection of fine membranous structures, such as the arachnoid membrane, could be accomplished with minimal effort and with significant precision. In addition, the wear and strength characteristics of silicon devices can be further enhanced through the use of thin film coatings of silicon nitride, which can be uniformly deposited on the shaped points and edges.
All of the aforementioned surgeon-enhancing strategies are of significant relevance to the minimally invasive surgery arena. The enhancement of precision and safety (e.g., the minimization of tremor) could significantly advance the field, which is currently severely limited by the surgeon’s physical limitations. Furthermore, MEMS and related microelectronics can create an environment for the development of enhanced workstations,
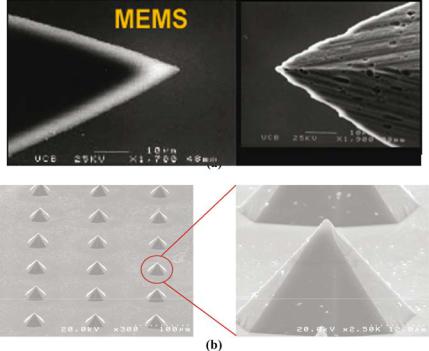
116 |
SHUVO ROY ET AL. |
FIGURE 6.16. SEM images of MEMS surgical sharps. (a) Sharper and smoother cutting edges are provided by the etching processes used in the fabrication of MEMS devices. (b) Array of piercing microprobes. (Courtesy: Verimetra Inc)
fly-by-wire technologies, and electronically enhanced tools that revolutionize the minimally invasive neurosurgical environment, which at the current time, is relatively stagnant.
6.7.5. In Vivo Spine Biomechanics
The application of MEMS to the spine arena is centered about its ability to monitor pressure, strains, and loads. Pressure within bone or within a cage, as well as strains and loads applied to interbody, ventral, and dorsal implants, are clearly of value to the researcher and the practicing surgeon. The telemetric transmission of this information to the treating physician could provide information that is indicative of fusion status, implant competence, and implant failure. A characteristic pattern of fusion and non-union most certainly exists on a clinical situation-specific basis as suggested in Figure 6.17. Such patterns are obviously speculative at this point. However, they are probably consistent within specific clinical applications. Furthermore, they most likely can differentiate fusion from non-fusion (pseudoarthrosis) status. Current methods for fusion assessment involve radiographic imaging techniques and the subjective assessment of patient pain. Conventional radiographic techniques, (CT, MRI, x-ray) provide only a snapshot in time of the condition [39]. Artifacts from the spinal instrumentation often disrupt the image and skew the clinical impression. In fact, the imaging of threaded interbody fusion constructs and other metallic implants often
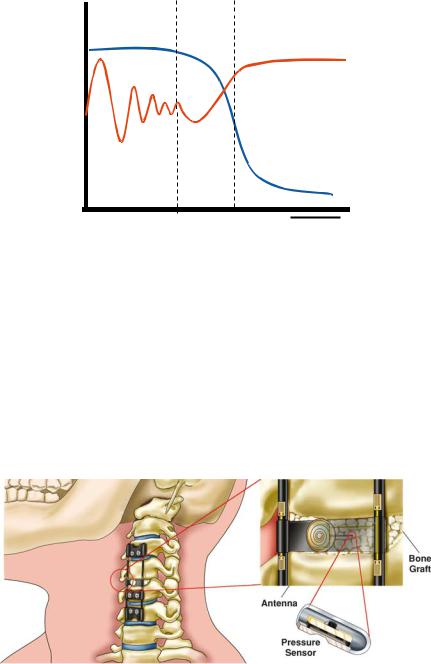
MEMS AND NEUROSURGERY |
117 |
Strain
Pressure
FUSION
IMPLANTATION
time
FIGURE 6.17. Graph showing possible relative variations in strains on spinal implants and pressure within bone graft versus time. The dashed lines depict the phases of fusion maturation.
cannot accurately display the bone-metal interface where the fusion is initiated [39]. Finally, patient derived information is highly subjective and a clinically accurate and complete history is not usually obtained.
The assessment of pressures, loads, and strains on an on-line basis via telemetric techniques permits the spine surgeon to assess biomechanical parameters in vivo. This forms the basis for the in vivo biomechanics laboratory.
Each patient with such monitoring capabilities inserted essentially is, in fact, such a laboratory. The in vivo biomechanics laboratory concept may be of much greater significance to the future of spine surgery than is intuitively apparent at the outset. Figure 6.18 presents the concept for a proposed fusion monitoring system based on implantable wireless
FIGURE 6.18. Schematic depiction of a concept for an implantable fusion assessment system using MEMS pressure sensors and strain gauges. The strain gauges monitor biomechanical loading of the cervical plate while the pressure sensor is used to assess pressure fluctuations within the bone graft. The measured pressure and load information is transmitted to an external receiver via wireless telemetry.
118 SHUVO ROY ET AL.
MEMS sensors that are mounted on spinal implants and within bone [38, 71]. The miniature size, integration with electronics, and manufacturability of MEMS sensors suggest that the corresponding fusion monitoring system could be readily transitioned into routine clinical practice for spine patients undergoing vertebral stabilization surgery. The combination of a MEMS sensor and a telemetric information transmission system can provide the surgeon with invaluable, otherwise unobtainable, data that is accurate, online, and real-time. This data is derived from a system (the combination of the patient and a MEMS-based implant) that requires no assumptions to generate the information. It only measures actual and realized parameters such as loads, strains, and pressures. Conversely, with traditional biomechanical laboratory testing or finite element modeling (FEM) studies, multiple assumptions are made. These include assumptions about paraspinous muscle activity and their contribution to stability, bone density, the applicability of the test performed to the clinical situation at hand, etc. If, in a given laboratory experiment, 10 such assumptions were made, and each was associated with a 20% chance of error (both are conservative estimates), a significant error would be introduced in the final analysis. This error, in fact, is portrayed by the predicted accuracy of such an experiment. If each assumption is associated with an 80% accuracy (20% chance of error), 10 assumptions would drop the accumulative accuracy to 0.810 = 0.107 = 10.7% accuracy [39].
In vivo biomechanical testing makes no assumptions. If the device or system is accurate, it simply provides in vivo, real-time information. If this can be routinely and readily achieved, the traditional biomechanics laboratory and finite element modeling strategies for spine applications will essentially become obsolete.
The implications to the treating physicians, as well as the scientist (e.g., engineer) and implant manufacturer regarding the strains and loads applied to a device, as well as the pressure within cages, is clearly of clinical, research, and marketing value. What such information permits is the in vivo monitoring of biomechanical parameters; hence the term “in vivo biomechanical testing” and the development of a new entity—the “in vivo biomechanics laboratory”.
6.7.6. Neural Regeneration
The miniaturization and electronics integration capabilities of MEMS technology may also be applicable to the development of interfaces for neural regeneration [43, 53]. Peripheral nerve injuries often recover since collagen and other structurally stabilizing substances are present in the peripheral nerve. This is not so in the central nervous system. Central nervous system regeneration may be facilitated by MEMS technology in four ways: [38] employment of hydraulic and micro-hydraulic principles to MEMS applications (microfluidics) via the employment of very small tubes to facilitate neural growth or the pumping of fluids; [39] facilitation of neural growth by the application of electrical potentials; [40] application of growth stimulation agents, such as growth factors, via MEMS driven drug delivery systems; and [41] utilization of silicon substrates for the attainment of spatially directed neuronal regeneration.
Figure 6.19 presents a proposed neuronal regeneration interface using MEMS technology [53]. The interface consists of a perforated silicon substrate that is designed to spatially constrain and direct the growth of regenerating nerve fibers from opposite directions. This design also includes metal electrodes that are patterned on the silicon substrate. These

MEMS AND NEUROSURGERY |
119 |
Electrode
Nerve Fiber
Microporous
Substrate
FIGURE 6.19. Schematic illustration of a MEMS-based neural regeneration interface. The perforated substrate spatially constrains the growing ends of the nerve fibers in close proximity. The electrodes are incorporated for recording and stimulation.
electrodes are incorporated in order to record axon signals and stimulate growth during the regeneration process.
Recent investigations into the use of MEMS technology for neural regeneration have focused on the development of hybrid neural interfaces, which incorporate a biological construct to enhance the integration of the synthetic implant into a host’s nervous system [48]. The biological constructs are typically either cells that outgrow from the interface into the host or neurophillic factors that coerce the host to grow into the interface [61, 63, 70, 74]. Figure 6.20 presents SEM images of a sieve electrode that incorporates proteins and nerve guide tubes to encourage axons to grow through the pores [63]. Nine recording
FIGURE 6.20. SEM images of a sieve electrode to encourage nerve cells to grow through the pores. (a) There are silicon leads (arrow) and nine iridium-coated active sites, which are 5–8 μm in diameter. (b) Close up of an 8 μm-diameter active site showing the iridium coating (arrow).
120 |
SHUVO ROY ET AL. |
sites are integrated via polysilicon leads into a silicon ribbon cable. The silicon leads are insulated from the external environment and internal diaphragm with dielectric layers.
6.8. PROSPECTS FOR MEMS IN NEUROSURGERY
The future of MEMS applications to the neurosurgical arena is immense. In fact, even the clinical employment of smart systems, the application of MEMS technology to the molecular biology arena, and the modification of cell growth via MEMS technology is imminently within reach. MEMS and related technologies can be utilized to enhance bone fusion, improve recovery following spinal cord and peripheral nerve injury, and for the management of patients with brain tumors and traumatic brain injury. The incorporation of MEMS devices into surgical robotics and navigation will enhance minimally invasive neurosurgery. Placement of sensors into operative tools for structural and physiological monitoring will minimize unnecessary damage to tissues while providing critical biological information. Ultimately, advances in the development of hybrid neural interfaces coupled with increased understanding at the cell/molecular biology level could lead to intriguing prospects for bionic integration [60]. What is readily achievable today is the monitoring of clinical parameters via minimally invasive means on a long-term basis [71].
The integration of MEMS technology into the medical milieu and the “neurosurgery milieu” faces many obstacles. Neurosurgeons must become more aware of the potential and limitations of MEMS technology. This effort will require educational initiatives that will bring together developers of MEMS technology with users in neurosurgery. For example, new training programs directed towards medical residents and research fellows will need to be established. In addition, comprehensive review articles and didactic textbooks must be written. The successful application of MEMS to the neurosurgical arena requires the merging of fields and disciplines, the merging of technologies and the collective gathering of expertise. Collegiality, communication, and significant investments will be required. Risks must be taken. However, the benefits derived will far outweigh the risk and the time and money invested.
ACKNOWLEDGEMENTS
We would like to thank David Schumick of the Department of Medical Illustrations at the Cleveland Clinic Foundation for his generous assistance with the original graphics that are extensively used throughout this article. We are also grateful to Dr. Michele Migliulo of Verimetra Inc., Pittsburgh, PA, and Dr. Jack W. Judy of the University of California, Los Angeles, for their generosity in providing illustrations and SEM images. In addition the following organizations are credited for our courtesy use of their graphics: Museum of Man (San Diego, CA), Medtronic Inc. (Minneapolis, MN), Elekta AB (Stockholm, Sweden), Guilford Pharmaceuticals Inc. (Baltimore, MD).
REFERENCES
[1]R. Bayston, W. Ashraf, and C. Bhundia. Mode of action of an antimicrobial biomaterial for use in hydrocephalus shunts. J. Antimicrob. Chemother., 53:778–782, 2004.