
Therapeutic Micro-Nano Technology BioMEMs - Tejlal Desai & Sangeeta Bhatia
.pdf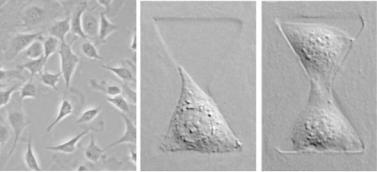
ENGINEERING CELL ADHESION |
|
327 |
A |
B |
C |
FIGURE 18.1. The figure illustrates three images of cells. A. a conventional cell culture where cell spreading and cell-cell contact are not regulated. B. a microfabricated cells culture system with constrained spreading and no cell-cell contacts and C. the same microfabricated cell culture system with constrained cell spreading and a single cell-cell contact. B and C are shown at a higher magnification than A (from [56]).
developed to meet those needs. Finally, we will describe areas of current and future work that are now emerging in the field of controlling cell adhesion, organization, and position.
18.2. REGULATING CELL FUNCTION VIA THE ADHESIVE MICROENVIRONMENT
Early in cell culture studies, it was found that cells in general require two classes of molecules—one is the class of basic metabolites such as ions and sugars, required for the building bocks of cells, and the other is that of signaling molecules, which can be both soluble and insoluble. Cells use signaling molecules, not directly as building blocks, but as elements of control systems that elicit appropriate changes in cell behavior in response to changes in the environment. For example, insulin is a soluble signaling protein secreted by the pancreas and forms a key element of the blood glucose control system in the body. Increases in blood glucose signal the release of insulin from the pancreas into the blood stream, and the subsequent regulation of blood glucose levels through a series of molecular events [23]. Cells sense their environment using different surface receptors, which bind specifically with different ligands.
In practice, biologists have experimentally manipulated soluble signals such as insulin simply by changing its concentration in the culture dish. However, cells also receive signals through adhesion receptors. Standard cell culture methods involve culturing cells, for example, in petri dishes. Changes in culture conditions, such as the ECM coating on the dish, are done at the level of the entire dish and not at the level of single cells. That is, all the cells are exposed to the same environment on the average. Experimentally manipulating cell-ECM adhesion, especially at single cell level, has been difficult with standard methods; methods were not available to localize the ECM at cell length scales. Because of the micrometer scale spatial control required, much of the progress made in the material science and semiconductor fabrication world has contributed to developing cell-patterning technologies. Before detailing these advances, we provide a brief background on current understanding of biological adhesion.
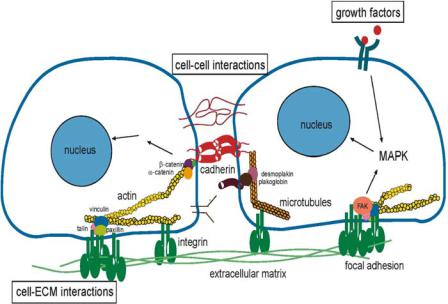
328 |
KIRAN BHADRIRAJU ET AL. |
FIGURE 18.2. The figure illustrates some of the molecules both within and outside cells involved in cell adhesion.
Cell adhesion is a well-studied mechanism of cell communication. Adhesion is a form of mechanical linkage, of cells to cells and cells to the ECM, and is critically involved in cellular signaling events that control proliferation, survival, apoptosis, shape, polarity, motility, and differentiation. Adhesion is mediated by transmembrane proteins, which connect the interior of the cell to its extracellular environment (Fig. 18.2). One major feature biological adhesion that is different from non-biological adhesion such as with household and industrial adhesives is that the former is mediated by chemical signals that often positively or negatively feedback to adhesion and subsequent cell behavior. In other words, cell adhesion is more than a simple glue to hold cells and tissues together; it is also a critical signaling platform. Integrins and cadherins are two principal classes of molecules mediating primarily cell-ECM and cell-cell adhesion respectively.
Integrins are the best-understood class of adhesion receptors [31]. They mediate both cell-ECM and cell-cell adhesion, differing based on the cell type and the type of receptor used in the interaction. Integrins, composed of α-β subunit heterodimers, assemble into 24 distinct integrins and bind to proteins in the ECM such as fibronectin, collagen and laminin [31]. The binding of integrins initiates clustering of integrins to form structures and the recruitment of a host of signaling and adaptor proteins as well as the actin cytoskeleton [24]. These focal adhesions are involved in the signaling events that lead to proliferation, motility, cytoskeletal organization, and cell survival [24].
Cadherins mediate adhesive contact between cells in structures called adherens junctions, and play a vital role in morphogenic events during development [83]. The best studied of these are the classical cadherins, which mediate adhesion between adjacent cells by forming homotypic junctions at sites called adherens junctions, and are linked intracellularly to

ENGINEERING CELL ADHESION |
329 |
the actin cytoskeleton through beta-catenin and alpha-actinin (Fig. 18.2). Similar to integrins, cadherins too have a mechanical and signaling role. Along with the mechanical that adhesion plays in tissue cohesion, cadherins, like integrins, transmit specific signals to the cell interior through proteins at the adhesion site [69, 85].
Both integrin and cadherin adhesions are biochemically regulated and are both dynamic and reversible. Normal cell processes such as the rounding of spread cells during mitosis, cell sorting and migration during embryogenesis, or disease processes such as cancer cell metastasis involve active changes in adhesion strength between cell-ECM and cell-cell contacts. Also, adhesive signals regulate many of the same cell functions that soluble growth factors do. Adhesion and growth factor pathways are cooperative: for example, anchorage dependent cells do not grow in the absence of adhesion when they are placed in suspension even in the presence of saturating amounts of growth factors, neither do they grow in the absence of growth factors even when adherent. It is only when both adhesive and growth factor signals are present that growth pathways are optimally activated in cells [89].
The variables involved in controlling cell-environment interactions to achieve a desired phenotype for tissue engineering may be summarized as in Fig. 18.3. The bold arrows
Similar |
Different |
Cells |
Cells |
ECM |
CELLS |
Growth |
|
Factors |
|||
|
|||
|
|
Microfabrication
Technology
Biological
Insights
FIGURE 18.3. The figure indicates the role that microfabrication technologies can play in advancing cell engineering. The block arrows indicate the path to achieving a desired cell phenotype by changing the different adhesive and soluble inputs that a cell receives from its environment. The thin arrows represent the iterative feedback processes of understanding and refinement of experiments towards this goal using microfabrication technologies.
330 |
KIRAN BHADRIRAJU ET AL. |
refer to possible inputs that each cell receives from the surroundings that are amenable to optimization by microfabrication technology. Such signals include adhesive interactions with the ECM, adhesion with similar neighboring cells (homotypic interactions), adhesion with dissimilar neighboring cells (heterotypic interactions), and signals from soluble growth factors in the environment. Researchers have demonstrated that each of these interactions is amenable to experimental control through microfabrication technology, as represented by the thinner arrows. Through iterative feedback of the resulting biological insights, it is thought that a desired phenotype can eventually be engineered explicitly with microsystems approaches.
18.3. CONTROLLING CELL INTERACTIONS WITH THE SURROUNDING ENVIRONMENT
Cell adhesion (to ECM and to other cells) is clearly important but is harder to control than soluble growth factor cues due to the difficulty in preparing defined surfaces. Most proteins are surface active due to the presence of hydrophobic and hydrophilic amino acids within their sequence and hence tend to accumulate at surfaces. This poses a challenge because cell culture media constituents such as serum are complex mixture of proteins, which differentially compete for adsorption to surfaces and leave a complex, ill-defined coating for experimental studies. The following section will describe two distinct technologies which have helped overcome the challenge of controlled cell adhesion: technologies that allowed preparing substrates with defined surface chemistry, and technologies that allowed the patterning of this surface chemistry on cell length scales.
18.3.1. Creating Defined Surface Chemistries
Traditional approaches to control surface chemistry have relied on pre-coating by adsorption of the culture surface with known concentrations of purified adhesion proteins such as fibronectin and subsequently blocking protein adsorptions to free surface areas with BSA (bovine serum albumin), which adsorbs well to hydrophobic surfaces. BSA repels further protein adsorption and is non-adhesive to cells. Using these methods to control cell adhesion and thereby spreading, researchers showed correlations between cell spreading and growth, differentiated function in microvascular endothelial cells [32] and hepatocytes [53]. However, this method to control cell adhesion suffered from the disadvantage that the physisorbed BSA is not stable, vulnerable to degradation by cell-derived proteases, and also necessitated the use of serum-free culture medium to minimize unwanted protein adsorption due to exchange with surface bound-BSA. Further, the methods did not allow varying local spatial organization of the ECM or the cells, as cells are randomly organized on the surface. Advances in surface engineering have allowed researchers to alleviate some of these limitations:
Several chemistries have been developed to immobilize proteins on activated surfaces using carboxy, amino, hydroxy, thiol, and other chemical moieties on amino acids [21]. For example, surface alcohols can be activated by substituting with the more reactive sulfonyl ester group, which can then be displaced by amino or thiol containing ligands [21]. Alternatively, light sensitive heterobifunctional bridges have been developed that link the
ENGINEERING CELL ADHESION |
331 |
substrate on one end and ligand on the other. For example, the photosensitive copolymer of styrene and vinylbenzyl N,N-diethyldithiocarbamate has been used to graft polymerize (N,N-dimethylacrylamide) onto poly(ethylene terephthalate) surfaces in the presence of UV light [55].
Besides protein immobilization, adhesion engineering also requires methods to block non-specific protein adsorption. This is achieved by localizing the blocking agent at the surface either by adsorption, or covalent coupling to alkanethiols or silanes. Hydrophilic polymers such as polyethylene oxide, polyethylene glycol, polyacrylamide, agarose, mannitol or the protein albumin have been used as blocking agents [36, 45]. Multiple factors appear to contribute to the non adhesivity of surfaces including the presence of surface charge, mobility of surface groups and ultimately the free energy cost of adsorption [3]. These chemistries have been critical to the development of surfaces with bio-specific adhesion, by eliminating non-specific, or undesired adhesion.
Self-assembled monolayers (SAMs) have been another important contribution to the development of defined surface chemistries. When certain long chained hydrocarbons are tethered to surfaces, they tend to present highly ordered surfaces due to thermodynamic and steric considerations [61]. The free end can be coupled to molecules of interest using suitable chemistries. Hence, surfaces can be functionalized by grafting a suitable end group on the free end of the molecules to engineer desired surface properties. For example, methyl terminated alkanethiols form extremely hydrophobic SAMs that permit protein adsorption whereas PEG-terminated (polyethylene glycol-terminated) alkanethiols form very hydrophilic surfaces that are resistant to protein adsorption [62]. Examples of physisorbed (i.e. not involving covalent bonding) SAMs include those prepared from Langmuir-Blodgett films (LB films) of synthetic double-tailed alkyl groups [59] and examples of chemisorbed (i.e. involving covalent bonding) SAMs include alkanethiolates on gold [62] and silanes on glass and silicon [47]. Alkanethiols and silanes have been extensively used for micropatterning and will be considered in further detail below.
Alkanethiols are hydrocarbons containing a sulfhydryl group on one end. When a solution of alkanethiols is exposed to gold, the sulfur atom forms covalent bonds with gold. Due to a combination of epitaxial registry with the underlying gold crystal structure and lateral stabilization of the hydrophobic alkyl chains, alkanethiols form highly oriented SAMs on gold [5], that can be exploited for engineering adhesion. The building blocks of silane SAMs are derivatives of the prototypical silane SiH4. One of the hydrogens attached to silicon is substituted with a long chain hydrocarbon terminating in a functional group such as an amine or a carboxyl group and the other three with alkoxy groups (-OR) or chlorine atoms. The alkoxy groups can be hydrolyzed in the presence of water or moisture to give silanols (-OH) which can further condense with similar groups on activated substrates as plasma treated glass or plastic, or amongst themselves, to create covalent crosslinks (O-Si-O). The amino or carboxy functional groups can be used for coupling of proteins [12, 47].
With these newfound controls over surfaces, researchers were able to study molecular aspects of cell adhesion previously not possible. Biologists had identified several recurring adhesion motifs in ECM proteins including the tripeptide RGD sequence found in a fibronectin, fibrinogen and collagens amongst others [67] and the YIGSR sequence in laminin [49]. The discovery of these specific adhesion sequences as parts of much larger proteins with multiple binding sites now allowed researchers to pose questions such as what
332 |
KIRAN BHADRIRAJU ET AL. |
the ligand density required for spreading is, or what the optimal molecular conformation is for receptor binding for specific ligands. Massia and Hubbell examined the question of the minimum density of ligand required for cell spreading and for focal adhesion formation [48]. Using covalent coupling of the integrin recognition sequence RGD to non adhesive glass surface, they reported that for human foreskin fibroblasts, the ligand spacing for maximal spreading was 440 nm and 140 nm for focal contact formation.
Adherent cells deposit their own matrix when plated on surfaces. Because of this, it was not known if short segments of ECM such as the RGD sequence were sufficient to maintain long-term cell health in the absence of augmentation by cell-deposited ECM. Roberts et al tested this question by making use of the exceptional protein repellent characteristics of oligoethyleneglycol-terminated thiols [66]. Surfaces created with RGD functionalized oligoethyleneglycol-terminated thiols (EG thiols) in a background of EG thiol were able to resist cell-secreted ECM deposition, and cells cultured on these surfaces survived for at least 24h [66]. They reported that microvascular endothelial cells were able to survive by attachment to RGD moieties in the absence of other proteins. A number of studies with immobilized RGD peptides had shown that RGD conformation determines its activity [52, 59]. Houseman et al examined the relation between RGD accessibility on a substrate and adhesion using the alkanethiol SAM system [28]. Using RGD terminated EG thiol in a bed of EG thiol of varying length (by changing the number of EG repeats, thereby changing the exposure of the RGD moiety), they showed by a variety of measures that cell responses such as spreading strongly depend on the background against which RGD is presented [28].
While these tools to present ECM ligands in better-defined systems have already provided important insights and advances to our understanding of a few key cell-surface adhesive interactions, the potential wealth of information to be gained from the much larger number of less well studied adhesive interactions remains untapped.
18.3.2. The Development of Surface Patterning
The technologies described so far allow uniform control of the adhesiveness of a surface. Generating surfaces with heterogeneous adhesiveness, where the pattern of adhesive and non-adhesive chemistries is prescribed, is called “patterning”. Patterning allows adhesive control at cell length scales. Patterning allows the ability to direct cell adhesion to desired regions of the substrate, exclude cells from other parts, and comprises the most basic level of spatial engineering of adhesion. Early efforts for micropatterning of cells used the deposition of palladium through a nickel mask, to make micrometer-sized adhesive squares of palladium on a non-adhesive background [15–16]. While significant insights into regulation of cell migration [16], neuronal guidance [43], and proliferation [81] were obtained using this method, it had the disadvantages of that mechanism of adhesion was not well defined and it required metal evaporation and specialized masks; hence, the techniques were not easily adaptable for general use by the biological research community.
Surface chemical modification using silanes and alkanethiols remedy some of these shortcomings and have been widely adopted for patterning cell adhesion to surfaces. Patterning with SAMs has involved coupling of the silane or alkanethiol to the surface by masking the remaining areas and then removing the mask [47], or selective removal of parts
ENGINEERING CELL ADHESION |
333 |
of a uniformly SAM-coated surface using UV ablation through a photomask [53]. However, these patterning tools have some disadvantages. For example, the materials used in the patterning process damage proteins and are cytotoxic. These limitations greatly impeded the mass production of patterned substrates. The development of microcontact printing alleviated some of these shortcomings.
Microcontact printing in its essence involves patterning regions with differing surface properties on a surface using physical stamps rather than direct lithographic approaches [70]. This patterning is achieved in the following manner. Photolithography is used to make a mold of the pattern of interest in a photoresist coated on a silicon wafer. A prepolymer of PDMS (polydimethylsiloxane) is then cured against this mold (‘master’) and peeled to reveal a PDMS ‘stamp’ containing a negative replica of the original master. The ‘inks’ used to stamp patterns on the gold surface are the silanes and alkanethiols [18, 82]. When the elastomeric stamp is placed in contact with a surface, the ink is transferred to it, forming a SAM wherever the stamp contacts the surface. Then a different silane or alkanethiol can be used to functionalize unstamped regions. In the end, the substrate now contains a pattern of two different SAMs. For patterning cells, chemistries are chosen such that one region is adhesive to ECM proteins and cells, while the other is resistant to protein adsorption, the latter typically being achieved by SAMs employing ethylene glycol functionalization. While microcontact printing with alkanethiols considerably lowered the barrier to micropatterning, it still requires equipment for gold coating of substrates and requires special chemicals such specially modified thiols and silanes. Direct printing of proteins might alleviate these dependencies by creating a general-purpose method to print proteins on suitable substrates, without the need for specialized equipment once a replicamolded stamp is available [73].
There have been several variations of surface patterning built around PDMS stamps molded from photolithographically generated ‘masters’, taking advantage the conformal contact with a flat surface. Using adjacent, parallel laminar flows in a PDMS channel sealed against a plastic dish, Takayama et al demonstrated the facility to pattern cells and their substrates using microfluidics [72]. If each channel contains a different protein for example, they will adsorb in parallel lines on the surface. In the absence of turbulent mixing between adjacent streams, the resolution of the technique essentially is determined by diffusion intermixing, which in turn is determined by the size of the particle being patterned. Using such devices, it has been shown possible to pattern cells over a uniformly coated surface [72], or partition the fluid phase over a single cell so that different parts of the cell are exposed to different aqueous environments [73]. Turbulence can be purposefully created to allow mixing when needed by patterning roughness on the channel walls [71]. Further refinements built around microfluidics patterning have allowed the aligned printing of multiple proteins on the same substrates [76] potentially enabling the study of cell interactions with more complex surface chemistries. These microfluidic approaches are described in greater detail in excellent reviews elsewhere [36–37].
18.3.3. Examples of Patterning-Based Studies on Cell-To-Cell Interactions
The tools for micropatterning described above have been used in several studies to examine the consequences of the organization of cells with respect to each other, and the resulting cell-to-cell interactions, on cell function. Some of these are described below.
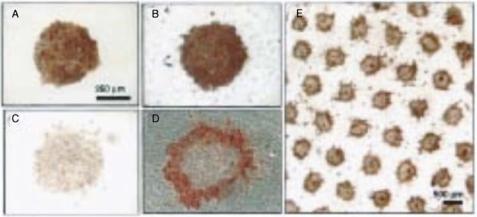
334 |
KIRAN BHADRIRAJU ET AL. |
FIGURE 18.4. The figure demonstrates the effect of introducing heterotypic cell-cell contacts on hepatocyte differentiation in vitro. Red color indicates the synthesis of albumin, a marker for hepatocyte differentiation. A and B respectively are micropatterned hepatocytes cultured in isolation or in co-culture with fibroblasts. C and D show cultures under the same conditions after six days. Note the greater loss of albumin from isolated hepatocytes C compared to co-cultures D. E shows a low-magnification image of the co-culture (from [8]).
18.3.3.1.Hepatocyte Differentiation In vivo, hepatocytes exist in heterotypic cellcell contact with other cells such as sinusoidal endothelial cells and stellate cells. Hepatocytes isolated from the liver rapidly lose their differentiated phenotype, by measures such as albumin or urea secretion [53]. As part of the effort to maintain hepatocytes differentiated function in vitro, investigators have tried to recapitulate the in vivo context. In this effort, it was found that introducing heterotypic cell-cell contact by co-culture of hepatocytes with fibroblasts increased hepatocytes differentiated function [40]. However, because these conventional cell culture experiments did not allow the modulation of cell-cell contact independent of cell number, it was difficult to ascertain the mechanism of the upregulated function [8]. By using micropatterning methods, Bhatia et al. were able to culture hepatocytes in a defined pattern and fibroblasts in the intervening regions, thus restricting cell-cell contacts to the interface between regions [7]. This allowed the investigators to increase cellcell contact at constant cell number and show that hepatocytes albumin synthesis is higher and is maintained for longer times with hepatocytes-fibroblast contact independent of cell density (Fig. 18.4). This study showed the potential for engineering enhanced differentiated function by using appropriate microfabricated substrates.
18.3.3.2.Angiogenesis The growth of the small capillary blood vessels in the body, or angiogenesis, underlies many important normal physiological and pathological events such as wound healing, diabetes, atherosclerosis and the growth of cancerous tumors [14]. Angiogenesis is also a key element of tissue engineering, as organ replacements will require the development of ways to populate the devices with blood vessels to cater to the oxygen and nutrient needs of the cells within the device [35]. Hence, it is of great medical interest to understand and control the process of angiogenesis. Researchers have attempted to coax microvascular endothelial cells in culture dishes to form branched network of tubular
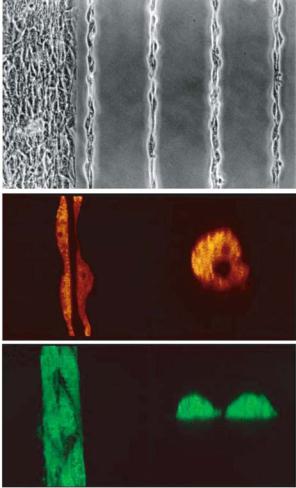
ENGINEERING CELL ADHESION |
335 |
A 10 μm
B 10 μm
C 30 μm
FIGURE 18.5. Microvascular endothelial cells cultured on 10 μm lines form tube-like structures. A. phase microscopy image of cells on 10 μm line. B. confocal sectioned image of the same cells showing a hollow lumen inside. C. cells on 30 μm lines which spread as ribbons and do not form a hollow lumen (from [20]).
vessels; enabling the study of the process in a convenient in vitro system. Conventional in vitro systems such as culturing endothelial cells on low densities of fibronectin [32] or within malleable gels of ECM [38], suffer from limitations such as a low yield of capillaries (low percentage of cells on the dish form capillaries) and random distribution disallowing convenient quantification of the process. Dike et al. have studied the role of the ECM and cell-cell contacts in angiogenesis by culturing endothelial cells on micropatterned lines of fibronectin [20]. While unspread endothelial cells apoptose in culture, cells cultured on wide (30 um) lines of ECM form extensive cell-cell contact but spread out as ribbons and do not form tubes (Fig. 18.5) [20]. Only cells cultured on an intermediate width (10 um) of fibronectin lines form both extensive cell-cell contacts and develop into tubular structures
336 |
KIRAN BHADRIRAJU ET AL. |
with a central lumen verified by microscopy [20]. Micropatterning angiogenesis models might in the future allow controlled studies of angiogenesis and in the design of substrates that promote angiogenesis in tissue engineered devices.
18.3.3.3. Cell Proliferation The previous study examined the relation between cellcell contact and cell shape on endothelial differentiation into capillaries. Nelson and Chen [56] examined the crosstalk between cell-cell contact and shape for endothelial proliferation using a novel patterning method that uses elements of microcontact printing (the use of a textured PDMS stamp) and microfluidic patterning (the use of flow though channels). The textured PDMS stamp is used to form channels against a glass surface while its elastomeric nature helps seal parts of the surface where the stamp is flush with it. This stamp was used as a mold to gel shallow wells of agarose on glass, within which pairs of cells were cultured (Fig. 18.1). The system allowed Nelson and Chen to independently control cell-cell contact and cell spreading. They used this system to demonstrate that cell-cell contact increases cell proliferation but that this effect is masked in conventional (unpatterned) cell culture conditions of unconstrained adhesion [56].
18.3.4.Examples of Patterning-Based Studies on Cell-Matrix Interactions
18.3.4.1.Cell Shape and Proliferation Some of the key biological problems addressed by micropatterning derive from an earlier study on the growth of cells on surface of graded adhesivity [22]. Substrate adhesivity to cells was changed by coating tissue culture surfaces with graded concentrations of the non-adhesive hydrogel poly(2-hydroxyethyl methacrylate). Cell spreading and correspondingly DNA synthesis (also termed ‘growth’) were similarly found to increase with increased density of fibronectin and collagen coating on surfaces [32, 53]. Because changing ECM density not only changes cell shape but also integrin signaling events such as integrin clustering and focal adhesion formation, it was not clear if the observed proliferative effects were due to changes in the integrin signaling as was conventionally understood, or due to cell shape itself acting as an input for the cell regulation.
Singhvi et al examined this question using microcontact printing [70]. By printings adhesive islands of the ECM protein laminin, of small or large areas, they could change cell shape without local ligand density. They found that indeed rounded hepatocytes on small squares exhibit low growth and high differentiated function while spread cells on large islands displayed the opposite [70]. This study strengthened the hypothesis that cell shape acts as a signal distinct from integrin clustering. However, because the ligand density was the same between round and spread cells, the total amount of ligand seen by the spread versus cells round was higher, which could by itself explain the increase proliferation in spread cells. Chen et al addressed this question, again by using microcontact printing technology [17]. This time, they varied cell shape while keeping the ECM density and ECM area constant. Instead of using single large islands of ECM to spread cells, they used arrays of small ECM dots which were spaced so that cells could bridge the gaps between the dots and thereby spread to eh same extent as cells on single large islands, although the total ECM that the cells came in contact was smaller in the case of dot patterns. This study showed that cell shape independent of the extent of ECM contact, modifies cell behavior. Huang et al extended this study by examining the effect of cell shape on some