
Therapeutic Micro-Nano Technology BioMEMs - Tejlal Desai & Sangeeta Bhatia
.pdfNANOPOROUS IMPLANTS FOR CONTROLLED DRUG DELIVERY |
285 |
[32]N. Jeandidier and S. Boivin. Current status and future prospects of parental insulin regimens, strategies and delivery systems for diabetes treatment. Advan. Drug Deliv. Rev., 35:179–198, 1999.
[33]E.M. Johnson, D.A. Berk, R.K. Jain, and W.M. Deen. Hindered diffusion in agarose gels: test of effective medium model. Biophy. J., 70:1017–23, 1996.
[34]C.G. Keller and M. Ferrari. Microfabricated particle filter. US Patent No. 5,651,900, 1997.
[35]C.G. Keller and M. Ferrari. High Vertical Aspect Ratio thin Film Structures, U.S. Patent No. 6,015,559, January18, 2000.
[36]C.G. Keller and M. Ferrari. Microfabricated Capsules for Immunological Isolation of Cell Transplants, US PatentNo. 5,893,974, April 13, 1999a.
[37]C.G. Keller and M. Ferrari. Microfabricated Particle Thin Film Filter and method of making it, U.S. Patent No.5948255, September 7, 1999b.
[38]A. Kikuchi and T. Okano. Pulsatile drug release using hydrogels. Advan. Drug Deliv. Rev., 54:53–77,2002.
[39]E.A. Klausner, S. Eyal, E. Lavy, M. Friedman, and A. Hoffman. Novel levodopa gastroretentive dosage form: in-vivoevaluation in dogs. J. Cont. Release, 88:117–126, 2003.
[40]V.M. Knepp, A. Muchnik, S. Oldmark, and L. Kalashnikova. Stability of nonaqueous supension formulations of plasma derived factor IX and recombinant human alpha interferon at elevated temperatures. Pharm. Res., 15(7):1090–1095, 1998.
[41]P.A. Krulevitch and A.W. Wang. Microfabricated Injectable Drug Delivery System. US Patent Appl. Publ. No. 2001044620, 2001.
[42]V. Kukla, et al. NMR studies of single-file diffusion in unidimensional channel zeolites. Science., 272:702– 704, 1996.
[43]L. Leoni, A. Boiarski, and T.A. Desai. Characterization of nanoporous membranes for immunoisolation: diffusion properties and tissue effects. Biomed. Microdevices., 4(2):131–, 2002.
[44]G.B. Lesinski, S. Sharma, K. Varker, P. Sinha, M. Ferrari, and W.E. Carson. Release of biologically functional interferonalpha from a nanochannel delivery system. Biomed. Microdev. (in press), 2004.
[45]D.G. Levitt. Dynamics of a single-file pore: non-fickian behavior. Phys. Rev. A (General Physics), 8:3050– 3054, 1973.
[46]J.R. Lewis and M. Ferrari. BioMEMS for drug delivery applications. In A. van den Berg (ed.) (Invited Chapter), Lab-on-a-Chip: Chemistry in Miniaturized Synthesis and Analysis Systems. pp. 101–115, 2003.
[47]B. Lin, J. Yu, and S. Rice. Direct measurements of constrained Brownian motion of an isolated sphere between two walls. Phys. Rev. E., 62:3909–19, 2000.
[48]J.M.D. MacElroy and S.H. Suh. Self-diffusion in single-file pores of finite length. J. Chem. Phys., 106:8595– 97, 1997.
[49]Z. Mao and S.B. Sinnott. A Computational study of molecular diffusion and dynamic flow through carbon nanotubes. J. Phys. Chem. B., 104:4618–4624, 2000.
[50]F. Martin, R. Walczak, A. Boiarski, M. Cohen, T. West, C. Cosentino, and M. Ferrari. Tailoring width of microfabricated nano-channels to solute size can be used to control diffusion kinetics. J. Control. Rel. (in press), 2004.
[51]T. Meersmann, et al. Exploring single-file diffusion in one-dimensional nanochannels by laser-polarized 129Xe NMR spectroscopy. J. Phys. Chem. A, 104:11665–11670, 2000.
[52]Z. Megeed, J. Cappello, J. Ghandehari. Genetically engineered silk-elastin-like protein polymers for controlled drug delivery. Adv. Drug Del. Rev., 54:1075–1091, 2002.
[53]B. Narasimhan and R. Langer. Zero-order release of microand macromolecules from polymeric devices: the role of the burst effect. J. Control. Rel., 47(1):13–20, July 7, 1997.
[54]P. Nelson and S. Auerbach. Self-diffusion in single-file zeolite membranes is Fickian at long times. J. Chem. Phys., 110:9235–9244, 1999.
[55]B.D. Ratner, A.S. Hoffman, F.J. Schoen, and J.E. Lemons (eds.). Biomaterials science. A Introduction to Materials in Medicine. Academic Press, San Diego, CA, 1996.
[56]J.H. Samet, H. Libman, K.A. Steger, et al. Compliance with zidovudine therapy in patients infected with humanimmunodeficiency virus, type 1: a cross-sectional study in a municipal hospital clinic. Am. J. Med., 92:495–502, 1992.
[57]J.T. Santini, M.J. Cima, and R. Langer. A controlled-release microchip. Nature, 397:335–338, 1999.
[58]J.T. Santini, M.J. Cima, and S.A. Uhland. Thermally-activated microchip chemical delivery devices. PCT Int. Appl., 2001.
286 |
TEJAL A. DESAI ET AL. |
[59]J.T. Santini, A.C. Richards, R. Scheidt, MJ. Cima, and R. Langer. Microchips as Controlled Drug-delivery Devices Angewandte Chemie, International Edition. 39:2396–2407, 2000.
[60]S. Sershen and J. West. Implantable, polymeric systems for modulated drug delivery. Adv. Drug Del. Rev., 54:1225–1235, 2002.
[61]S. Sharma, K.C. Popat, and T.A. Desai. Controlling non-specific protein interactions in silicon biomicrosystems withpoly(ethylene glycol) films. Langmuir, 18(23):8728–8731, 2002.
[62]P. Sinha, G. Valco, S. Sharma, X. Liu, and M. Ferrari. Nanoengineered device for drug delivery application. Nanotechnology, 15:S585–S589, 2004.
[63]A. Spiess, V. Mikalunas, V. Carlson, M. Zimmer, and R.M. Craig. Albumin kinetics in hypoalbuminemic patients receiving total parenteral nutrition. J. Parenter. Enter. Nut., 20:424–428, 1996.
[64]B. Spilker. Methods of assessing and improving patient compliance in clinical trails. In B. Spilker (ed.), Guide toClinical Trials. New York, Raven Press, pp. 102–114, 1991.
[65]R.K. Stoelting. Pharmacokinetics and pharmacodynamics of injected and inhaled drugs. In Pharmacology andPhysiology in Anesthetic Practice. Lippincott-Raven, New York, pp. 3–35, 1999.
[66]S.L. Tao, and T.A. Desai. Microfabricated drug delivery systems: from particles to pores. Adv. Drug Del.Rev., 55:315–328, 2003.
[67]F. Theeuwes and S.I. Yum. Principles of the design and operation of generic osmotic pumps for the delivery of semisolid or liquid drug formulations. Ann. Biomed. Eng., 4:343–353, 1976.
[68]J.K. Tu and M. Ferrari. Microfabricated Filter and Capsule Using a Substrate Sandwich. U.S. Patent No. 5,938,923, Aug. 17th, 1999.
[69]J.K. Tu, T. Huen, R. Szema, and M. Ferrari. Filtration of sub-100nm particles using a bulk-micromachined, direct-bonded silicon filter. Biom. Microdev., 1(2):113–120, 1999.
[70]R. Walczak, A. Boiarski, T. West, J. Shapiro, S. Sharma, M. Ferrari. Long-term biocompatibility of NanoGATE drug delivery implant. Nanobiotechnology (invited paper, submitted) 2004.
[71]Q. Wei, C. Bechinger, P. Leiderer. Single-file diffusion of colloids in one-dimensional channels. Science, 287:625–27, 2000.
[72]N. Wisniewski, B. Klitzman, B. Miller, and W.M. Reichert. Decreased analyte transport through implanted membranes: Differentiation of biofouling from tissue effects. J. Biomed. Mater. Res., 57:513–521, 2001.
[73]R.C. Wood, E.L. LeCluyse, and J.A. Fix Assessment of a model for measuring drug diffusion through implantgenerated fibrous capsule membranes. Biomaterial., 16(12):957–959, 1995.
[74]J.C. Wright, S.T. Leonard, C.L. Stevenson, J.C. Beck, G. Chen, R.M. Jao, P.A. Johnson, J. Leonard, and R.J. Skowronski. Anin vivo/in vitro comparison with a leuprolide osmotic implant for the treatment of prostate cancer. J. Control. Rel., 75:1–10, 2001.
[75]T. Yasukawa, H. Kimura, Y. Tabata, and Y. Ogura. Biodegradable scleral plugs for vitreoretinal drug delivery. Adv. Drug Del. Rev., 52(1):25–36, Oct. 31, 2001.
III
Molecular Surface Engineering for the Biological Interface
16
Micro and Nanoscale Smart
Polymer Technologies in
Biomedicine
Samarth Kulkarni, Noah Malmstadt, Allan S. Hoffman, and Patrick S. Stayton
Department of Bioengineering, University of Washington, Seattle, WA 98195
Polymers that exhibit a sharp hydrophilic to hydrophobic phase transition on the application of an environmental stimulus such as pH or temperature are called smart polymers. These smart polymers, or stimuli responsive polymers, have been used to develop several drug delivery technologies. The emergence of the fields of nanotechnology and microfluidics has created new opportunities for smart polymers. We have recently developed two nanoand microscale technologies for diagnostic applications. The first is a reversible particle system using stimuli-responsive polymer-protein conjugates. We have found that conjugates of streptavidin and the temperature-responsive poly(N-isopropylacrylamide) (PNIPAAm), rapidly form stable and uniformly sized mesoscale particles above the lower critical solution temperature (LCST) of the polymer. The size of these particles is dependent on concentration, molecular weight of the polymer used and formulation parameters such as the heating rate. The second is a stimuli-responsive bioanalytical bead system. Latex beads were dualconjugated with PNIPAAm and an affinity ligand to confer temperature-responsiveness to the beads. Above the LCST of the PNIPAAm, the bead surface becomes hydrophobic and the modified beads aggregate and adhere to the walls of microfluidic channels. They have been used to develop a reversible microfluidic affinity chromatography matrix for the upstream processing of complex fluids and for immunoassays. Both technologies can be used in a wide variety of formats, including microfluidic-based micro-total analytical systems (μTAS) devices and simple, rapid field tests.
290 |
SAMARTH KULKARNI ET AL. |
16.1. SMART POLYMERS
The ‘smartness’ of smart polymers is derived from their rapid, reversible and highly cooperative response to simple physical stimuli, such as temperature, pH, or light. Much like highly cooperative biological reactions such as protein folding, smart polymers respond with a property change of large magnitude in response to very small changes in environmental conditions. Upon application of the stimulus, the stimuli-responsive polymers ‘switch’ from a hydrophilic, expanded state to a hydrophobic collapsed state, a change that can be manifested in one of many forms. Free polymers might switch from a solvated state in aqueous solution to a phase separated state. Cross-linked hydrogels display swelling or shrinking in response to stimuli, and surfaces with smart polymers exhibit a dramatic change in wettability. All these changes are reversible upon restoration of the original conditions.
Since the publication of the first work on the ‘smart’, temperature sensitive polymer PNIPAAm in 1968 [1], there have been more than 15000 publications in the area of smart polymers. The biggest impact of smart polymers has been in the biomedical field, where the versatility and usefulness of these polymers as molecular engineering tools has been demonstrated in the development of biological sensors, drug delivery vehicles and tissue engineering [2–4]. More recently, the unique properties have been employed in the nanoand microscale space, to develop useful biosensors and bioanalytical technology. This chapter will primarily focus on the use of smart polymers in micro/nanotechnology based biosensors, diagnostics and therapeutics. For a broader review of smart polymers, readers are directed to other reviews of the literature [5, 6].
16.1.1. Mechanism of Aggregation
The molecular engineering of “biohybrid” materials composed of stimuli-responsive polymers and biomolecules can take several forms. Polymer chains can be covalently end-attached or grafted to individual protein, lipid or DNA molecules, or the polymer could be used to modify the surface of a synthetic nanoparticle that is also modified with biomolecules. These materials exhibit a smart response to stimuli, similar to that the original isolated polymer, though some interesting alterations in the polymer response can also be generated and exploited. We have demonstrated the conjugation of smart polymers to proteins, antibodies, oligonucleotides, plasmid DNA, lipids, ligands such as biotin and several synthetic drug molecules for use in drug delivery, molecular separations, and diagnostics [2, 7–10].
PNIPAAm is one of the most extensively studied and utilized smart polymers, with a rich 35 year history after first being reported in 1968 [1]. PNIPAAm exhibits a lower critical solution (LCST) behavior. Below a certain temperature designated as the LCST, it is hydrophilic and highly solvated, while above the LCST, it is aggregated and phase separated. On a macroscopic level, this change is observed as the development of turbidity in a solution of PNIPAAm, which can be measured by a change in its transmittance. Of great interest is the sharp transition from individual chains to the aggregated state over a very narrow temperature range of a few degrees. The change is completely reversible and reversal of the stimulus results in the PNIPAAm going back into solution rapidly. Various techniques have been used to determine the LCST of PNIPAAm including UV turbidimetry, IR spectroscopy, light scattering, calorimetry and fluorescence techniques
MICRO AND NANOSCALE SMART POLYMER TECHNOLOGIES IN BIOMEDICINE |
291 |
[11–14]. Recently, a microfluidic system with a temperature-gradient has been developed by the Cremer and coworkers to measure the LCST of thermosensitive polymers [15].
The mechanism and energetics of the PNIPAAm phase transition have been extensively characterized [16–18]. PNIPAAm is fully solvated below the LCST, with water ordering around the isopropyl surface, along with a complex hydrogen bonding network between water molecules and the polymer hydrogen bond acceptor and donor atoms. Below the LCST, the favorable enthalpy associated with the hydrogen bonding network is sufficient to drive the highly solvated state of the polymer. Chain collapse above the LCST is entropically driven by water release to the higher entropy state of bulk solvent, where the T S entropy term becomes favorable compared to the H enthalpic contribution to the free energy. The energetics of the PNIPAAm phase separation have been previously reviewed [19].
On a molecular level, the mechanistic events following the elevation of the temperature above the LCST have also been characterized using light scattering techniques. Using high molecular weight chains (> 106 g/mol) at dilute concentrations, collapse of individual chains of PNIPAAm above the LCST to form thermodynamically stable globules was observed. The water released from the fully solvated polymer chain results in the rapid collapse of the polymer, determined by measuring the radius of gyration by Wu et al. [20]. A schematic model for the post-stimulus phase separation behavior of PNIPAAm is shown in Figure 16.1. At these dilute concentrations (picomolar), chain collapse is followed by interchain association and nucleation of globule clusters that subsequently grow in size through a poorly controlled aggregation process, as evidenced from an increase in the polydispersity of particle size. Kinetic studies have shown that these small globules are formed rapidly after application of the stimulus and also disaggregate within a minute. The aggregate sizes are heterogeneous and depend on formulation parameters (e.g. heating rate) and PNIPAAm molecular weight (number-averaged, Mn). Experiments with mixed polymer systems in a microfluidic device with a temperature gradient have shown that the initial formation of particles is kinetically controlled, followed thermodynamic rearrangement [15]. With dynamic light scattering experiments, we have found that for lower Mn PNIPAAm and concentrations in the nanomolar to picomolar range, kinetically-controlled formation of small hydrophobic globules is followed by further aggregation over time to produce large, non-uniform particles [21].
16.2. SMART MESO-SCALE PARTICLE SYSTEMS
16.2.1. Introduction
The unique properties of nanoscale particles have been exploited to develop new biotechnology applications by coupling the particles to biomolecules [22–25]. Nanoparticles modified with oligonucleotides have been used to detect DNA targets, and proteinmodified nanoparticles have been used in drug delivery [26, 27]. Polymers have often been used as building blocks for these particles. For example, block copolymers of hydrophilic poly(ethylene oxide) and hydrophobic poly(ethylethylene) have been used to self-assemble micelles, cylindrical rods and vesicles in aqueous phases [28]. The bottom-up strategy used in making these particles exploits the hydrophobic effect as a driving force for self-assembly [29].
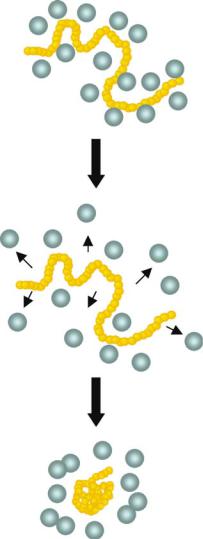
292 |
SAMARTH KULKARNI ET AL. |
FIGURE 16.1. Temperature sensitive polymer, PNIPAAm, is fully solvated below the LCST of the polymer. When the solution is heated to temperatures above the LCST, PNIPAAm becomes hydrophobic, and the water around PNIPAAm is disordered. Finally, the polymer collapses and intrachain aggregation is seen within seconds.
We have developed a particle system in which PNIPAAm, in its hydrophobic state, directs assembly of polymer-protein conjugates into particles of controlled sizes. By using a smart polymer, the reversible hydrophilic to hydrophobic phase transition of smart polymers can be exploited to develop a reversible particle system in the nanoand mesoscale size range. When a solution of PNIPAAm is heated above its LCST, it transitions to a hydrophobic state that is driven by partial dehydration. The PNIPAAm-biomolecule conjugates in this state exhibit a nucleation and growth process into particles with narrow size polydispersity.
MICRO AND NANOSCALE SMART POLYMER TECHNOLOGIES IN BIOMEDICINE |
293 |
On reversal of the stimulus, the particles redissolve rapidly to the free conjugate species. The different properties of the free versus particulate species can then be exploited to reversibly capture and release target analytes, turn enzymes on and off, and reversibly adhere targets to device surfaces.
16.2.2. Preparation of PNIPAAm-Streptavidin Particle System
The synthesis of PNIPAAm by reversible addition fragmentation chain transfer (RAFT) polymerization provides precise control over the molecular weight of the polymer [30, 31]. The RAFT technique allows the synthesis of polymers with very narrow molecular weight distribution, which is important in determining the effect of molecular weight on particle size. Previous studies have shown that in a polydisperse mixture of PNIPAAm polymers, the higher molecular weight polymers phase separate first followed by the smaller polymers [17, 20]. Studies by Mao et al. have, however, shown that the polydispersity of the polymer solution does not affect the LCST [15]. The effect of molecular weight on particle size is not clear, although preliminary results indicate that for bioconjugate particle formation, the molecular weight has to be greater than a threshold level. Another advantage of RAFT is that it yields PNIPAAm with a reactive sulfhydryl containing end-group, which can then be modified using any of several commercially available sulfhydryl-reactive biotin molecules, such as the iodoacetyl biotin or maleimide biotin.
The initial characterization of the stimuli-responsive particles was conducted on molecular conjugates of streptavidin and PNIPAAm. The PNIPAAm molecule is first biotinylated at the end group using maleimide conjugation chemistry and then mixed with streptavidin to form a PNIPAAm-streptavidin complex. Because the four biotin binding sites of streptavidin are located in proximal pairs on opposite faces of the streptavidin tetramer, steric constraints limit the complexation of the biotinylated PNIPAAm chain to one site per face.
16.2.3. Mechanism of Aggregation
Below the LCST, the polymer and the protein are both hydrophilic and the conjugate remains in solution. Above the LCST, however, the bioconjugate undergoes an interesting transition into an amphiphile-like conjugate, with streptavidin as the hydrophilic block and the PNIPAAm as the hydrophobic block. This drives the aggregation of the conjugate into uniform sized particles as depicted in Figure 16.2. We have previously observed that PNIPAAm nucleates and grows over time into continuously larger aggregates in aqueous solution above the LCST [21]. It was also seen that in the presence of surfactant, the aggregates are more stable and uniform sized [13, 32]. Similar to the effect of surfactants, particles formed from the biohybrid conjugates are stabilized by the charged and hydrophilic streptavidin, and remain stable even at higher concentrations. The protein thus serves as a hydrophilic capping agent to limit the size of the bioconjugate particle.
16.2.4. Properties of PNIPAAm-Streptavidin Particle System
The most remarkable feature of this particle system is the rapid and reversible dissolution of the particles. A study of the kinetics of particle formation and growth revealed that stable particles formed within a minute of stimulus application. For PNIPAAm alone,

294 |
SAMARTH KULKARNI ET AL. |
FIGURE 16.2. The mechanism of mesoglobule formation by PNIPAAm is shown. In panel A, when a solution of PNIPAAm is heated above the LCST, the polymer collapses. This is followed by interchain aggregation to form particles with wide distribution. PNIPAAm globules keep on aggregating resulting in unstable particles. In panel B, when PNIPAAm-streptavidin conjugate is heated, the polymer collapses and directs aggregation with other conjugates. However, after initial aggregate formation, the globules do not aggregate further due to the surfactant-like effect of streptavidin.
the aggregates formed in two stages: initial rapid aggregation into uniform sized particles within one minute, followed by continuous further aggregation of the particles. For the streptavidin-PNIPAAm molecules, there was no further aggregation after the initial rapid aggregation due to the presence of the hydrophilic streptavidin on the surface of the particles. Another striking feature is the control over the particle size by various ‘engineering handles’. The particle size was seen to be strongly dependent on the concentration of the conjugate, molecular weight of the polymer used, the stimulus applied and the rate of application of the stimulus. By manipulating these features of the conjugate system, the particle size could be ‘tuned’ between 200 and 900 nm for the PNIPAAm-streptavidin system.
16.2.5. Protein Switching in Solution using Aggregation Switch
An interesting property of the protein-polymer particle system is the effect of particle formation on the activity of the protein. By engineering precisely the site of attachment of the polymer onto an enzyme surface, the activity of the enzyme could be turned on or off in the particle state. If the polymer was attached at a position away from the active site, the enzyme was active in both the free and particle state. However, if the polymer was attached
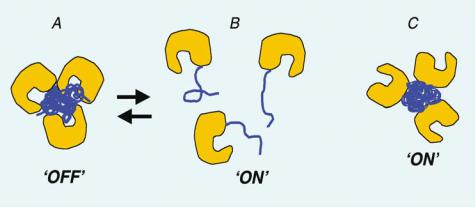
MICRO AND NANOSCALE SMART POLYMER TECHNOLOGIES IN BIOMEDICINE |
295 |
FIGURE 16.3. Enzyme endoglucanase is conjugated at various sites to temperature and light sensitive polymer, PDMAAm. When the PDMAAm is conjugated close to the active site of the enzyme, the aggregate formed on heating occludes the access of the substrate to the binding site. If the PDMAAm would be conjugated away from the active site, it would form a biofunctional particle on heating above the LCST or providing the appropriate light stimulus. Thus the ‘aggregation switch’ can be controlled site-specifically.
near the active site, the enzyme was active in the free state, but turned off in the particle state. This suggested that the orientation of the enzyme active site could be controlled in the particle such that substrate binding was allowed or blocked, depending on the orientation of the polymer relative to the active site (Figure 16.3). This application was demonstrated with both thermal and photo-responsive polymers that served as molecular switches to control the activity of the commercially important enzyme, endoglucanase 12A [2, 33, 34].
A unique photoand temperature-responsive smart polymer, poly(N, N- dimethylacrylamide-co-N-4-phenylazophenylacrylamide) (PDMAAm) was synthesized using free-radical polymerization. Normally, the polymer poly(dimethylacrylamide) has an intrinsic LCST in aqueous solutions well above 100 ◦C. By copolymerizing the dimethylacrylamide (DMA) with the hydrophobic phenylazophenyl monomer, the LCST of the polymer was reduced to 37 ◦C. By illuminating the polymer with far UV light at approximately 360 nm, a cis to trans change in configuration at the azo groups is induced. This configuration change affects the LCST of the polymer, shifting it to 43 ◦C. The ratio of DMA to phenylazophenyl monomer can be manipulated to tune the LCST properties such that an optimal temperature for a given enzyme can be selected where photo-stimulation leads to reversible expansion and collapse of the polymer chain. Furthermore, the LCST values are also affected by the ionic strength of the solution, providing a system with several control levers.
As illustrated in Figure 16.3 (configuration A), a site on the endoglucanase enzyme surface was chosen to be near the binding pocket groove. A surface exposed asparagine residue (N55) was chosen and mutated using recombinant DNA techniques to a cysteine that contains a sulfhydryl group. Using vinyl sulfone functionalized DMAAm, the polymers were conjugated to the endoglucanase. The switching of the polymer was demonstrated using different stimuli, temperature and light, and in both, immobilized or free solution formats. When immobilized on the beads, a molecular switching phenomenon occurs,