
Bioregenerative Engineering Principles and Applications - Shu Q. Liu
..pdf686 VASCULAR REGENERATIVE ENGINEERING
vascular injury and cell death. These changes facilitate blood coagulation, thrombogenesis, and intimal hyperplasia, resulting in arterial restenosis.
To reduce stent-induced vascular disorders, materials with β and γ radiation or antiproliferative drug coating, have been used to construct vascular stents. Stents with radiation materials can induce DNA damage and cell arrest in the G1 phase. Such stents have been shown to inhibit smooth muscle cell proliferation and prevent intimal hyperplasia after stenting surgery. However, radiation may induce cell death and vascular aneurysm. The control of radiation dosage is a critical issue. Antiproliferative drug-coated stents are effective for preventing smooth muscle proliferation, thus reducing intimal hyperplasia and stent failure.
Arterial Reconstruction. Vascular grafts are often used to replace malfunctioned arteries due to atherosclerosis. A number of materials have been developed and used for clinical or experimental arterial reconstruction. These materials include autogenous arteries and veins, allogenic arteries, non-biodegradable polymers (polytetrafluoroethylene and Dacron), biodegradable polymers (polyglycolides and polylactides), and extracellular matrix constituents (collagen matrix, fibrin matrix, and elastic laminae). Among these grafting materials, the arterial and vein grafts are considered the gold standard grafts because of their high patency rate and long lifespan following arterial reconstruction. However, not all patients possess arteries and veins available for arterial reconstruction. Allogenic vascular grafts and synthetic polymers have been used for arterial reconstruction. Although these materials exhibit characteristics suitable for arterial reconstruction, they induce inflammation and thrombogenesis, contributing to arterial restenosis. Further research is necessary to improve the anti-inflammatory and antithrombotic properties of these materials. Compared to angioplasty and stenting, arterial reconstruction has been proven a more effective approach for the treatment of atherosclerosis.
Arterial reconstruction models are often used in vascular tissue regenerative engineering to test new materials and assess the interaction of materials with host cells and tissues. This type of model can be created in a number of animals, including nonhuman primates, pigs, dogs, rabbits, rats, and mice. To establish such a model, an animal can be anesthetized by peritoneal injection of sodium pentobarbital (50 mg/kg). The artery of interest can be exposed and a segment of the artery is surgically separated from surrounding tissue and clamped with vascular clamps to stop local blood flow at two locations: one proximal and the other distal to the reconstruction site. The selected artery is transected between the two clamps. The lumen of the transected artery is immediately treated with 100 U/mL heparin. A segment of the host artery can be removed with its length depending on the length of the arterial graft. The arterial graft can be anastomosed to the host artery by using disrupted suture stitches. Note that continuous stitches often cause arterial constriction at the anastomosis. After anastomotic procedures, the vascular clamps can be released slowly. When bleeding occurs at the anastomoses, the artery can be reclamped and the bleeding sites can be sealed with additional suture stitches. After vascular clamps are removed, the artery ought to be inspected for blood flow by observing pulse activities. The surgical wounds can be closed in the order of the muscular tissue, soft connective tissue, and skin. Continuous suture stitches can be used to close the muscular and connective tissues, but discontinuous stitches are necessary for skin closure, since animals, especially rodents, intend to chew the suture stitches.
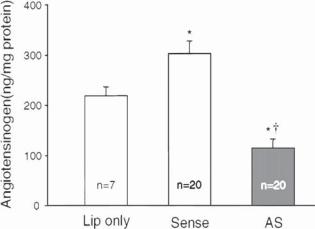
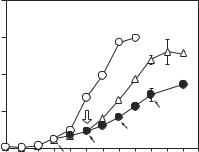
688 VASCULAR REGENERATIVE ENGINEERING
vascular smooth muscle cells and prevent intimal hyperplasia in vascular injury and graft models. Antisense oligonucleotides for E2F mRNA have also been used in clinical trials of human vein grafts. Preliminary studies have shown promising results.
Cell Cycle Inhibiting Genes [15.11]. The progression of cell cycle is negatively regulated by a number of inhibiting proteins, including the p53, p21CIP1, p27KIP1 (see page 265 for characteristics and regulation of these proteins). The genes encoding these inhibitory proteins can be prepared and used for transferring into arterial lesion sites. The overexpression of these genes has been shown to inhibit vascular cell proliferation and intimal hyperplasia in animal models.
Nitric Oxide Synthase Gene [15.12]. Nitric oxide synthase is an enzyme that catalyzes the deamination of L-arginine to produce nitric oxide. Nitric oxide can readily diffuse through the cell membrane, reacts with guanylyl cyclase to produce cyclic GMP (cGMP), and potentially inhibits the activity of cyclin A, a molecule that regulates cell mitosis. In experimental investigations, nitric oxide has been shown to effectively inhibit the proliferation of vascular smooth muscle cells (Fig. 15.11). Furthermore, nitric oxide enhances the expression and activity of the cell cycle-inhibiting protein p21CIP1, inhibits cell proliferation, and suppresses platelet aggregation and leukocyte adhesion. The enhancement of nitric oxide production in vascular cells leads to the suppression of cell proliferation. Since nitric oxide synthase is a limiting factor for the production of nitric oxide, the overexpression of this gene via gene transfer enhances the production of nitric oxide.
Characteristics of several nitric oxide synthases are listed in Table 15.5.
|
200 |
|
|
|
|
|
|
|
|
|
|
(× 104/well) |
150 |
|
|
|
|
|
|
control |
|
|
|
|
|
|
|
|
|
|
|
|
wash |
||
|
|
|
|
|
|
|
|
|
|
||
100 |
|
|
|
|
|
|
|
|
|
|
|
number |
|
|
|
|
|
|
|
|
|
|
|
|
|
|
|
|
|
|
|
|
|
SNAP |
|
50 |
|
|
|
|
|
|
|
* |
|
|
|
Cell |
|
|
|
|
|
|
|
|
|
||
|
|
|
|
|
|
|
* |
* |
|
|
|
|
|
|
|
|
* |
* |
|
|
|
||
|
|
|
|
|
|
|
|
|
|||
|
|
|
|
|
|
|
|
|
|
||
|
0 |
|
|
|
|
|
|
|
|
|
|
|
|
|
|
|
|
6 |
7 |
8 |
9 |
10 11 |
|
|
0 |
1 |
2 |
3 |
4 |
5 |
|||||
|
|
|
|
|
|
Day |
|
|
|
|
Figure 15.11. Influence of nitric oxide on vascular smooth muscle cell (VSMC) proliferation. The effect of S-nitroso-N-acetylpenicillamine (SNAP, a nitric oxide-releasing substance) on VSMC proliferation. VSMCs seeded in 6-well plates (3 × 104/well) were cultured in growth medium (open circle). The medium was changed every other day and the cells were counted every day. Two-thirds of the cultures (solid circle) were exposed to SNAP (100 μM) added every other day simultaneously with the changing medium (filled arrows). On day 5 (open arrow), one-half of the cultures treated with SNAP were placed in SNAP-free growth medium after several washes (triangle), and the other half were continuously exposed to SNAP. Data represent the means ± SD (n = 3). *p < 0.01 versus control. (Reprinted with permission from Ishida A et al: Induction of the cyclin-dependent kinase inhibitor p21(Sdi1/Cip1/Waf1) by nitric oxide-generating vasodilator in vascular smooth muscle cells, J Biol Chem 272:10050–7, copyright 1997.)


690 VASCULAR REGENERATIVE ENGINEERING
Herpes Virus Thymidine Kinase Gene [15.13]. Viral thymidine kinase can phosphorylate nucleosides and nucleoside substitutes. When viral thymidine kinase is present together with a nucleoside substitute, such as ganciclovir, the thymidine kinase can phosphorylate the nucleoside substitute, forming nucleoside triphosphate. The substitute nucleotide can be incorporated into DNA during DNA replication. Once the substitute nucleosite is integrated into a DNA molecule, DNA replication is terminated because the substitute nucleosite can no longer bind to additional nucleotides. Thus, a treatment with Herpes virus thymidine kinase gene and ganciclovir inhibits DNA replication and cell proliferation (Fig. 15.12).
Dominant Negative Mutant Mitogenic Genes [15.14]. Mitogenic genes encode proteins which regulate cell proliferative activities. Proteins encoded by dominant negative mutant genes may lose their mitogenic function, but keep their binding capability. When such dominant negative genes are expressed, their protein products can compete with the physiological form of the same proteins for substrate proteins that regulate cell mitogenic activities and thus partially block the substrate proteins, resulting in reduced mitogenic activities. A typical example of such dominant negative mutant genes is rasN17. This mutant gene is generated by replacing the gene codon for a serine residue at the 17th amino acid with a codon for asparagine. The RasN17 protein can competitively bind to substrate proteins, such as mitogen activated protein kinase kinase kinases, which are critical protein kinases for the regulation of cell mitogenic activities. The binding of RasN17 does not activate the substrate proteins, but block their interactions with the physiological form of Ras, thus reducing mitogenic activities (Fig. 15.13).
Vascular Tissue Regenerative Engineering. Vascular tissue regenerative engineering is to reconstruct blood vessels with cell-integrated vascular substitutes and to improve
Figure 15.12. Effect of ganciclovir (GC) on intimal and medial areas in arteries after balloon injury and infection with ADV-tk vector. Representative cross sections from iliofemoral arteries of pigs (A) injured for 1 min, then infected with adenoviral (ADV)- thymidine kinase (tk) vector and treated with saline (left) or ganciclovir (right); (B) injured for 5 min, then infected with ADV-tk vector and treated with saline (left) or ganciclovir (right); (C) injured for 5 min, then infected with ADV-δ E1 and treated with saline (left) or ganciclovir (right). These arteries were examined 3 weeks after injury and gene transfer (hematoxylin and eosin stain magnification 87×). Measurements of I/M area ratios (D) are from arteries infected after a 1-min injury and 3 weeks posttransfection with ADV-tk vector and treated with saline (0.445 +/− 0.047, n = 4 arteries) or ganciclovir (0.057 +/− 0.027, n = 4 arteries); infected after a 5-min injury and 3 weeks posttransfection with ADV-tk vector and treated with saline (0.512 +/− 0.047, n = 8 arteries) or ganciclovir (0.205 +/− 0.065, n = 8 arteries); infected after a 5-min injury and 6 weeks posttransfection with ADV-tk vector and treated with saline (0.639 +/− 0.099, n = 4 arteries) or ganciclovir (0.334 +/− 0.024, n = 4 arteries); infected after a 5-min injury and 3 weeks posttransfection with ADV-δ E1 vector and treated with saline (0.481 +/− 0.020, n = 8 arteries), or ganciclovir (0.445 +/− 0.027, n = 8 arteries). A statistically significant reduction in I/M area ratios was observed in the ADV-tk/positive GC group compared with ADV-tk/negative GC (1 min, 3 weeks, two-tailed unpaired t test, P < 0.05); ADV-tk/positive GC compared with ADV-tk/negative GC, ADV-δ E1/negative GC, and ADV-δ E1/positive GC (5 min, 3 weeks, ANOVA with Dunnett’s t test, P < 0.05); and ADV-tk/posi- tive GC compared with ADV-tk/negative GC (5 min, 6 weeks, two-tailed unpaired t test, P < 0.05). I, intima; M, media; GC, ganciclovir. (Reprinted with permission from Ohno T et al: Science 265:781–4, copyright 1994 AAAS.)
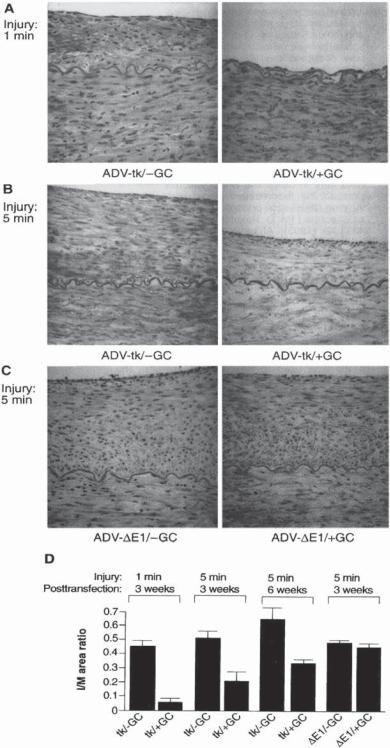
VASCULAR DISORDERS |
691 |
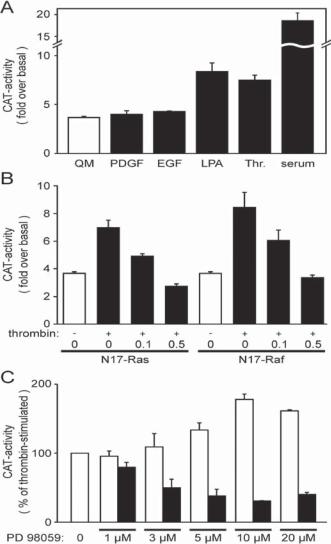
Figure 15.13. Negative regulation of extracellular signal-regulated kinase (ERK)1/2 phosphorylation by N17-Ras and N17-Raf. Receptor-mediated activation of the smooth muscle (SM) –myosin heavy-chain (MHC) promoter requires the Ras/Raf/ERK signaling cascade. Panel A, Vascular smooth muscle cells (VSM) cells, serum-starved for 48 h, were transfected with a 1346-nucleotide SM-MHC promoter-CAT fusion construct (pCAT-1346) and then incubated in the presence of serum free medium (QM) or in QM supplemented with 10 ng/mL PDGF, 10 ng/mL EGF, 10 μM LPA, 1 unit/mL thrombin (Thr.), or 10% serum for another 48 h. Cells were lysed and assayed for CAT activity. Depicted CAT activities were normalized for protein concentrations and compared with the CAT activity of cells transfected with a reporter gene construct lacking the SM-MHC promoter. Bars represent the means ± SE of at least five independent transfection experiments. Panel B, to test for participation of Ras/Raf in the thrombin-mediated SM-MHC promoter induction, VSM cells were cotransfected with 0.5 μg/well pCAT-1346 and the indicated amounts (in μg) of dominant negative N17-Ras or N17-Raf expression constructs. The total amount of plasmid DNA was kept constant (1 μg/well) with promoterless pCAT-basic. Panel C, VSM cells transfected with 1 μg/ml pCAT-1346 were preincubated for 30 min with different concentrations of PD98059, an inhibitor for mitogen activated protein kinase/ERK kinase (MEK black bars), and then stimulated with 1 unit/mL thrombin. Because dimethyl sulfoxide (the solvent of PD98059) further enhanced thrombin-stimulated SM-MHC promoter activities, controls were incubated in equivalent concentrations of the solvent (open bars). All values were normalized to the thrombin-mediated CAT activity without PD98059. (Reprinted with permission from Reusch HP et al: Gbeta gamma mediate differentiation of vascular smooth muscle cells, J Biol Chem 276:19540–7, 2001 with permission.)
VASCULAR DISORDERS |
693 |
the performance of reconstructed blood vessels. A number of approaches have been developed and used for engineering blood vessels. These approaches are discussed as follows.
Construction of Polymeric Arterial Substitutes with Endothelial Cell Seeding [15.15].
Polymeric materials have long been used for constructing arterial substitutes. Common polymeric materials used for vascular reconstruction include polytetrafluoroethylene (PTFE, Teflon) and polyethylene terephthalate (PET, Dacron). However, these materials induce inflammatory reactions and thrombogenesis, resulting in arterial restenosis and occlusion. Thus, polymeric materials can be used only for the reconstruction of blood vessels with diameter larger than 4 mm. To resolve such a problem, endothelial cells have been used to seed polymeric arterial substitutes on the luminal surface. While the approach seems reasonable, it is difficult to retain endothelial cells on the substitute during and after the reconstruction surgery. Major factors that influence the retention of endothelial cells include surgical trauma and bloodflow-induced shear stress. Further investigations are necessary to improve the polymeric surface properties and to enhance the retention of endothelial cells.
Construction of Cell-Integrated Arterial Substitutes with Biodegradable Polymers [15.16]. Another strategy in vascular tissue regenerative engineering is to construct biodegradable polymeric vascular substitutes with integrated vascular endothelial cells on the luminal surface and smooth muscle cells within the wall of the substitute. Several types of biodegradable polymeric materials, including polyglactin, poly-L-lactic-co- epsilon-caprolactone, poly(glycolide-co-caprolactone, polydioxanone, polyglycolic acid, and polyhydroxyoctanoates, have been used and tested for such a purpose. It is expected that, with the gradual degradation of polymeric materials, implanted cells within the materials can proliferate and produce extracellular matrix, self-generating a functional arterial substitute. While experimental results are promising, there are potential problems. First, implanted cells may not be able to generate an arterial substitute with the structural and mechanical properties of natural arteries. With the degradation of the polymeric materials, the arterial substitutes may exhibit reduced mechanical strength, inducing arterial aneurysm and rupture. Second, it is difficult to retain implanted endothelial cells. Third, polymeric materials, once subject to blood, induce blood coagulation and thrombosis, resulting in intimal hyperplasia and arterial restenosis. It is necessary to resolve these problems before biodegradable polymeric materials can be used for arterial reconstruction.
Construction of Arterial Substitutes with Extracellular Matrix [15.17]. Extracellular matrix components serve as substrate for cell attachment and growth, and determine the strength and elasticity of blood vessels. Thus, these components have long been considered materials for arterial reconstruction. Allogenic extracellular matrix is readily available and is often used for such a purpose. Because allogenic cells induce immune rejection reactions, matrix tissues are usually decellularized before being applied to arterial reconstruction. The extracellular matrix components exhibit significantly lower immunogenicity compared to cellular components. The implantation of matrix-based vascular constructs does not cause severe acute immune rejection responses.
Several types of extracellular matrix have been used for the construction of arterial substitutes. These include allogenic arterial matrix scaffolds, intestinal submucosa, and dermal collagen matrix. These are mostly collagen-based connective tissues characterized by natural matrix structure and suitable mechanical properties such as stiffness, elasticity,
694 VASCULAR REGENERATIVE ENGINEERING
and strength. Experimental investigations have shown that collagen matrix-based arterial substitutes can serve as substrates for the attachment and migration of vascular endothelial and smooth muscle cells. These substitutes can eventually integrate into the host arteries, forming naturalized arteries. However, collagen matrix components stimulate blood cell adhesion, coagulation, thrombogenesis, smooth muscle cell proliferation and migration, and intimal hyperplasia. These are pathological changes that cause substitute restenosis and failure.
Elastin-containing structures, including elastic fibers and laminae, are major extracellular matrix constituents. These structures contribute to the elasticity, stiffness, and strength of blood vessels. In particular, large elastic arteries contain multiple elastic laminae, which are essential for the maintenance of the stability of arteries. Furthermore, elastin-containing matrix exerts an inhibitory effect on inflammatory reactions, leukocyte adhesion and activation, thrombosis, smooth muscle cell proliferation, and intimal hyperplasia. In vitro studies have demonstrated that arterial elastic lamina specimens induce significantly lower monocyte adhesion and activation compared to arterial adventitial collagen matrix. In vivo studies in animal models have shown that arterial grafts with an elastic lamina-based blood-contacting surface exhibit significantly lower thrombosis and intimal hyperplasia compared to arterial grafts with a collagen matrix-based blood contacting surface (Fig. 15.14). These inhibitory features render elastic laminae a potential material for constructing the blood contacting surface of arterial substitutes.
The inhibitory role of arterial elastic laminae is mediated by a cell signaling pathway, which involves the inhibitory receptor signal-regulatory protein α (SIRPα) and SH2 domain-containing protein tyrosine phosphatase 1 (SHP1). The interaction of monocytes with elastic laminae induces autophosphorylation of SIRPα and recruitment of SHP1 to the cytoplasmic domain of SIRPα. This process activates SHP1, which in turn dephosphorylates several potential protein tyrosine kinases, including receptor tyrosine kinases, the Src family protein tyrosine kinases, phosphatidylinositol 3-kinase, and the Janus family tyrosine kinases. The dephosphorylation of these protein tyrosine kinases results in the suppression of inflammatory and mitogenic responses. These preliminary studies have provided promising results for the use of elastic laminae as a blood-contacting material for arterial reconstruction.
Construction of Arterial Substitutes with Decellularized Arterial Matrix Scaffolds Seeded with Endothelial Progenitor Cells [15.17] Bone marrow endothelial progenitor cells can be mobilized to the blood and are present in the blood at a basal level under physiological conditions. These cells can be isolated from blood samples based on their adherent properties and expression of endothelial progenitor markers. Isolated endothelial progenitor cells can be expanded by cell culture in vitro, and used to seed decellularized arterial matrix scaffolds, which can be used for arterial reconstruction. As shown in an experimental investigation, while the matrix scaffold-based arterial substitutes without endothelial progenitor cell seeding failed within 15 days, the arterial substitutes with endothelial progenitor cell seeding remained patent for 130 days. Furthermore, the arterial substitutes with endothelial progenitor cell seeding exhibited contractile function compared to the control arterial substitutes without endothelial progenitor cell seeding. These observations suggest that bone marrow-derived endothelial progenitor cells can be potentially used for improving the performance of reconstructed arteries.
Construction of Arterial Substitutes in vivo [15.18]. Cells and tissues are capable of initiating inflammatory reactions and producing extracellular matrix in response to the
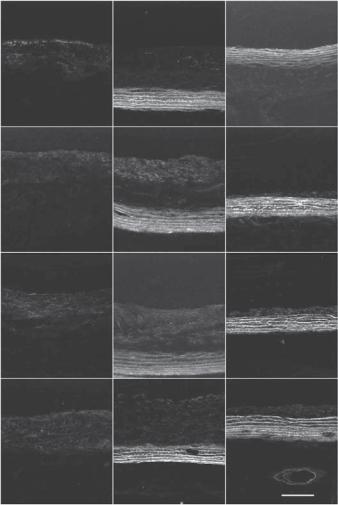
|
|
VASCULAR DISORDERS |
695 |
Vein grafts |
Adventitia |
Elastic laminae |
|
5 days
10 days
20 days
30 days
Figure 15.14. Matrix-based arterial reconstruction with an elastic lamina blood-contacting surface. Fluorescent micrographs showing anti-smooth muscle (SM) α actin antibody-labeled SM cells in matrix-based rat aortic constructs. Note that the neointimal layer on the elastic lamina bloodcontacting surface is apparently thinner than that on matrix-based constructs with a collagen surface and vein grafts. Arrow: blood-contacting surface. Scale: 100 μm.
stimulation of foreign implants of various materials, including polymers, metals, and allogenic and xenogeneic biological tissues. Such features can be used to generate arterial substitutes in vivo. When a cylindrical tube or rod is implanted into a soft connective tissue (e.g., subcutaneous tissue), muscular tissue, or the abdominal cavity, the implant can stimulate inflammatory reactions, resulting in the formation of a layer of encapsulating tissue, containing various types of cells, such as macrophages, fibroblasts, and epithelial cells, and extracellular matrix composed of collagen fibers and proteoglycans. The encapsulating tissue can be removed from the cylindrical implant and used as an arterial