
книги студ / color atlas of physiology 5th ed[1]. (a. despopoulos et al, thieme 2003)
.pdf
|
|
! |
|
|
|
|
united to form a close electrical and metabolic |
||
|
|
unit (syncytium), as is present in the |
||
|
|
epithelium, many smooth muscles (single- |
||
|
|
unit type, !p. 70), the myocardium, and the |
||
|
|
glia of the central nervous system. Electric |
||
|
|
coupling permits the transfer of excitation, |
||
|
|
e.g., from excited muscle cells to their adjacent |
||
Physiology |
|
cells, making it possible to trigger a wave of ex- |
||
|
citation across wide regions of an organ, such |
|||
|
as the stomach, intestine, biliary tract, uterus, |
|||
|
|
|||
|
|
ureter, atrium, and ventricles of the heart. Cer- |
||
|
|
tain neurons of the retina and CNS also com- |
||
Cell |
|
municate in this manner (electric synapses). |
||
|
Gap junctions in the glia |
(!p. 338) and |
||
|
|
|||
and |
|
epithelia help to distribute the stresses that |
||
|
occur in the course of transport and barrier ac- |
|||
Fundamentals |
|
tivities (see below) throughout the entire cell |
||
|
|
|||
|
|
community. However, the connexons close |
||
|
|
when the concentration of Ca2+ (in an extreme |
||
|
|
case, due to a hole in cell membrane) or H+ |
||
|
|
concentration increases too rapidly ( !C3). In |
||
1 |
|
other words, the individual (defective) cell is |
||
|
left to deal with its own problems when neces- |
|||
|
|
|||
|
|
sary to preserve the functionality of the cell |
||
|
|
community. |
|
|
|
|
Transport through Cell Layers |
|
|
|
|
|
||
|
|
Multicellular organisms have cell layers that |
||
|
|
are responsible for separating the “interior” |
||
|
|
from the “exterior” of the organism and its |
||
|
|
larger compartments. The epithelia of skin and |
||
|
|
gastrointestinal, urogenital and respiratory |
||
|
|
tracts, the endothelia of blood vessels, and neu- |
||
|
|
roglia are examples of this type of extensive |
||
|
|
barrier. They separate the immediate extra- |
||
|
|
cellular space from other spaces that are |
||
|
|
greatly different in composition, e.g., those |
||
|
|
filled with air (skin, bronchial epithelia), |
||
|
|
gastrointestinal contents, urine or bile |
||
|
|
(tubules, |
urinary bladder, |
gallbladder), |
|
|
aqueous humor of the eye, blood (endothelia) |
||
|
|
and cerebrospinal fluid (blood–cerebrospinal |
||
|
|
fluid barrier), and from the extracellular space |
||
|
|
of the CNS (blood–brain barrier). Nonetheless, |
||
|
|
certain substances must be able to pass |
||
|
|
through these cell layers. This requires selec- |
||
|
|
tive transcellular transport with import into |
||
|
|
the cell followed by export from the cell. Un- |
||
|
|
like cells with a completely uniform plasma |
||
18 |
|
membrane |
(e.g., blood cells), epiand en- |
|
|
dothelial cells are polar cells, |
as defined by |
||
|
|
their structure (!p. 9A and B) and transport function. Hence, the apical membrane (facing exterior) of an epithelial cell has a different set of transport proteins from the basolateral membrane (facing the blood). Tight junctions (described below) at which the outer phospholipid layer of the membrane folds over, prevent lateral mixing of the two membranes (!D2).
Whereas the apical and basolateral membranes permit transcellular transport, paracellular transport takes place between cells. Certain epithelia (e.g., in the small intestinal and proximal renal tubules) are relatively permeable to small molecules (leaky), whereas others are less leaky (e.g., distal nephron, colon). The degree of permeability depends on the strength of the tight junctions (zonulae " occludentes) holding the cells together (!D). The paracellular pathway and the extent of its permeability (sometimes cation-specific) are essential functional elements of the various epithelia. Macromolecules can cross the barrier formed by the endothelium of the vessel wall by transcytosis (!p. 28), yet paracellular transport also plays an essential role, especially in the fenestrated endothelium. Anionic macromolecules like albumin, which must remain in the bloodstream because of its colloid osmotic action (!p. 208), are held back by the wall charges at the intercellular spaces and, in some cases, at the fenestra.
Long-distance transport between the various organs of the body and between the body and the outside world is also necessary. Convection is the most important transport mechanism involved in long-distance transport (!p. 24).
Despopoulos, Color Atlas of Physiology © 2003 Thieme
All rights reserved. Usage subject to terms and conditions of license.

C. Gap junction |
|
|
|
|
|
|
|
|
II |
COO– |
Cell 1 |
|
Channel |
Cells |
|
|
|||
|
|
|
(1.2–1.5 nm) |
|
|
|
|
and Between |
|
NH3+ |
Cell 2 |
Cytosol 1 |
||
|
|
|
||
|
Cell membranes |
|
|
|
|
|
|
Cytosol 2 |
In, Through |
|
|
|
|
|
|
1 |
|
Ions, ATP, cAMP, |
1.9 Transport |
|
|
|
amino acids, etc. |
|
2 |
3 |
|
|
|
Connexon of Cell1 |
|
|
||
Connexin |
|
|
||
Connexon of Cell 2 |
|
|
Plate |
|
(27kDa) |
|
|
||
|
|
|
||
|
Channel open |
Channel closed |
|
D. Apical functional complex |
|
|
|
1 |
Apical |
Microvilli |
|
|
|
||
|
|
Tight |
Para- |
|
|
junction |
cellular |
|
See (2) |
|
transport |
|
|
|
|
Cell 2 |
Actin- |
|
|
Zonula |
|
myosin |
|
Cell 1 |
belt |
|
|
adherens |
|
||
|
|
|
|
Epithelial cells (e.g., enterocytes) |
Basolateral |
|
N |
|
|
|
|
|
|
|
N |
|
|
Occlusin |
|
|
Myosin II |
E-cadherin |
|
|
|
|
|
Actin |
|
Adapter proteins |
Ca2+ |
|
|
|
|
2 |
|
|
19 |
|
|
|
Photos: H. Lodish. Reproduced with permission from Scientific American Books, New York, 1995.
Despopoulos, Color Atlas of Physiology © 2003 Thieme
All rights reserved. Usage subject to terms and conditions of license.

Passive Transport by Means of
Diffusion
|
Diffusion is movement of a substance owing to |
|||||
|
the random thermal motion (brownian move- |
|||||
|
ment) of its molecules or ions (!A1) in all |
|||||
|
directions throughout a solvent. Net diffusion |
|||||
Physiology |
or selective transport can occur only when the |
|||||
solute concentration at the starting point is |
||||||
|
||||||
|
higher than at the target site. (Note: uni- |
|||||
|
directional fluxes also occur in absence of a |
|||||
|
concentration gradient—i.e., at equilibrium— |
|||||
Cell |
but net diffusion is zero because there is equal |
|||||
flux in both directions.) The driving force of |
||||||
and |
diffusion is, therefore, a concentration gra- |
|||||
|
||||||
Fundamentals |
dient. Hence, diffusion equalizes concentra- |
|||||
tion differences and requires a driving force: |
||||||
|
||||||
|
passive transport (= downhill transport). |
|||||
|
Example: When a layer of O2 gas is placed |
|||||
|
on water, the O2 quickly diffuses into the water |
|||||
|
along the initially high gas pressure gradient |
|||||
1 |
(!A2). As a result, the partial pressure of O2 |
|||||
(Po2) rises, and O2 can diffuse further |
||||||
|
||||||
|
downward into the next O2-poor layer of water |
|||||
|
(!A1). (Note: with gases, partial pressure is |
|||||
|
used in lieu of concentration.) However, the |
|||||
|
steepness of the Po2 profile or gradient (dPo2/ |
|||||
|
dx) decreases (exponentially) in each sub- |
|||||
|
sequent layer situated at distance x from the |
|||||
|
O2 source (!A3). Therefore, diffusion is only |
|||||
|
feasible for transport across short distances |
|||||
|
within the body. Diffusion in liquids is slower |
|||||
|
than in gases. |
|
|
|
||
|
The diffusion rate, Jdiff (mol·s–1), is the |
|||||
|
amount of substance that diffuses per unit of |
|||||
|
time. It is proportional to the area available for |
|||||
|
diffusion (A) and the absolute temperature (T) |
|||||
|
and is inversely proportional to the viscosity |
|||||
|
(η) of the solvent and the radius (r) of the dif- |
|||||
|
fused particles. |
|
|
|
||
|
According to the Stokes–Einstein equation, |
|||||
|
the coefficient of diffusion (D) is derived from T, |
|||||
|
η, and r as |
|
|
|
||
|
D ! |
R ! T |
|
[m2 ! s–1], |
[1.1] |
|
|
NA · 6π ! r |
! η |
||||
|
|
|
|
|||
|
where R is |
the general |
gas constant |
|||
|
(8.3144 J·K–1 · mol–1) and NA Avogadro’s con- |
|||||
|
stant (6.022 · 1023 mol–1). In Fick’s first law of |
20 |
diffusion (Adolf Fick, 1855), the diffusion rate |
|
is expressed as |
||
|
Jdiff ! A ! D ! ! |
dC |
"[mol ! s–1] |
[1.2] |
dx |
where C is the molar concentration and x is the distance traveled during diffusion. Since the driving “force”—i.e., the concentration gradient (dC/dx)—decreases with distance, as was explained above, the time required for diffusion increases exponentially with the distance traveled (t #x2). If, for example, a molecule travels the first µm in 0.5 ms, it will require 5 s to travel 100 µm and a whopping 14 h for 1 cm.
Returning to the previous example (!A2), if the above-water partial pressure of free O2 diffusion (!A2) is kept constant, the Po2 in the water and overlying gas layer will eventually equalize and net diffusion will cease (diffusion equilibrium). This process takes place within the body, for example, when O2 diffuses from the alveoli of the lungs into the bloodstream and when CO2 diffuses in the opposite direction (!p. 120).
Let us imagine two spaces, a and b (!B1) containing different concentrations (Ca "Cb) of an uncharged solute. The membrane separating the solutions has pores x in length and with total cross-sectional area of A. Since the pores are permeable to the molecules of the dissolved substance, the molecules will diffuse from a to b, with Ca –Cb = C representing the concentration gradient. If we consider only the spaces a and b (while ignoring the gradients dC/dx in the pore, as shown in B2, for the sake of simplicity), Fick’s first law of diffusion
(Eq. 1.2) can be modified as follows:
Jdiff ! A ! D ! |
C |
[mol ! s–1]. |
[1.3] |
|
x |
||||
|
|
|
In other words, the rate of diffusion increases as A, D, and C increase, and decreases as the thickness of the membrane ( x) decreases.
When diffusion occurs through the lipid membrane of a cell, one must consider that hydrophilic substances in the membrane are sparingly soluble (compare intramembrane gradient in C1 to C2) and, accordingly, have a hard time penetrating the membrane by means of “simple” diffusion. The oil-and-water partition coefficient (k) is a measure of the lipid solubility of a substance (!C).
!
Despopoulos, Color Atlas of Physiology © 2003 Thieme
All rights reserved. Usage subject to terms and conditions of license.
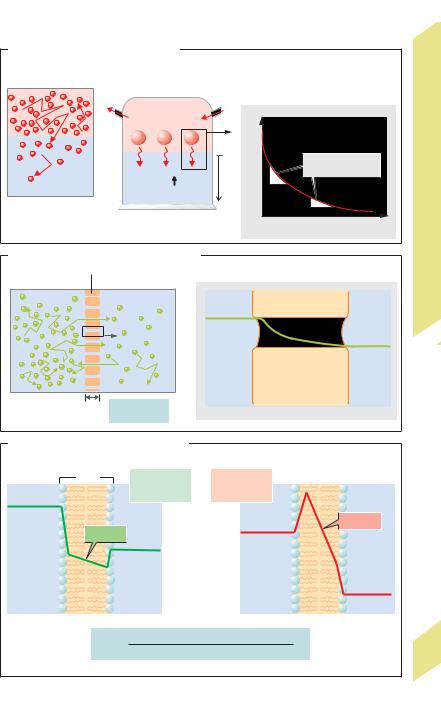
A. Diffusion in homogeneous media |
|
|
|
|
|
||
1 Brownian particle |
|
|
|
|
|
|
I |
|
|
Gas |
O2 |
|
|
Diffusion |
|
movement (~T) |
|
|
|
|
|||
2 |
Passive transport |
|
3 |
PO2 profile |
|
||
|
|
|
|
|
|
||
|
|
PO2 |
|
|
|
|
of |
|
|
|
|
|
|
|
|
|
O2 |
O2 |
O2 |
|
PO2 |
|
Means |
|
|
|
|
0 |
|
|
|
|
|
|
|
|
Slope=gradient |
by |
|
|
|
|
|
|
|
||
|
|
|
|
X |
P |
=dP/dx |
|
|
|
|
|
Transport |
|||
|
|
PO2 |
|
|
|||
|
|
|
|
|
x |
||
|
|
|
|
|
|
||
|
|
|
Water |
|
|
|
|
|
|
|
|
|
|
|
|
|
|
|
|
|
|
P |
|
|
|
|
|
|
|
x |
|
|
|
|
|
|
0 |
Distance from O2 source (x) |
Passive |
B. Diffusion through porous membranes |
|
|
|
1 |
Porous membrane |
2 |
|
1.10 |
||
|
|
|
|
|
|
|
Space a |
|
Space b |
|
|
Plate |
|
|
|
|
|
Ca |
|
|
Ca |
|
|
Cb |
Pore |
Gradient |
Cb |
|
|
|
|
|
|
|
|
x |
|
|
Space a |
Membrane |
Space b |
|
Ca–Cb= C |
|
|
|
||
|
|
|
|
|
||
C. Diffusion through lipid membranes |
|
|
|
|||
|
|
|
1 |
2 |
|
|
|
5nm |
|
Hydrophilic |
Hydrophobic |
|
|
|
|
|
substance X |
substance Y |
|
|
CXa |
|
|
(k <1) |
(k >1) |
|
|
|
Gradient |
|
CYa |
|
Gradient |
|
|
CXb |
|
|
|
||
|
|
|
|
|
|
|
|
Lipid |
|
|
|
Lipid |
CYb |
Water |
Water |
|
|
|||
membrane |
Water |
membrane |
Water |
|||
|
|
k = |
Equilibrium concentration in olive oil |
|
||
|
|
|
Equilibrium concentration in water |
|
21 |
|
|
|
|
|
|
(Partly after S.G.Schultz) |
Despopoulos, Color Atlas of Physiology © 2003 Thieme
All rights reserved. Usage subject to terms and conditions of license.

!
The higher the k value, the more quickly the substance will diffuse through a pure phospholipid bilayer membrane. Substitution into Eq. 1.3 gives
|
Jdiff ! k ! A ! D ! |
|
C |
[mol ! s–1]; |
[1.4] |
|||||||||||
|
|
|
||||||||||||||
|
|
|
|
|
|
|
|
|
|
x |
|
|
|
|
||
|
Whereas the molecular radius r (!Eq. 1.1) still |
|||||||||||||||
|
largely determines the magnitude of D when k re- |
|||||||||||||||
Physiology |
mains constant (cf. diethylmalonamide with ethyl- |
|||||||||||||||
urea in D), k can vary by many powers of ten when r |
||||||||||||||||
|
||||||||||||||||
|
remains constant (cf. urea with ethanol in D) and can |
|||||||||||||||
|
therefore have a decisive effect on the permeability |
|||||||||||||||
|
of the membrane. |
|
|
|
|
|
|
|
||||||||
Cell |
|
Since the value of the variables k, D, and |
x |
|||||||||||||
within the body generally cannot be deter- |
||||||||||||||||
and |
||||||||||||||||
mined, they are usually summarized as the |
||||||||||||||||
|
||||||||||||||||
Fundamentals |
permeability coefficient P, where |
|
|
|||||||||||||
P ! k ! |
D |
[m ! s–1]. |
|
|
[1.5] |
|||||||||||
|
|
|
|
|
||||||||||||
|
|
|
|
|
x |
|
|
|
|
|
|
|
|
|||
|
If the diffusion rate, Jdiff [mol!s–1], is related to |
|||||||||||||||
|
area A, Eq. 1.4 is transformed to yield |
|
|
|||||||||||||
1 |
|
Jdiff |
|
! P ! |
C [mol ! m–2 ! s–1]. |
[1.6] |
||||||||||
|
|
|
||||||||||||||
|
|
A |
|
|||||||||||||
|
|
|
|
|
|
|
|
|
|
|
|
|
|
|
||
|
The quantity of substance (net) diffused per |
|||||||||||||||
|
unit area and time is therefore proportional to |
|||||||||||||||
|
C and P (!E, blue line with slope P). |
|
||||||||||||||
|
|
When considering the diffusion of gases, |
C |
|||||||||||||
|
in Eq. 1.4 is replaced by α· |
P (solubility coeffi- |
||||||||||||||
|
cient |
|
times |
partial |
pressure. difference; |
|||||||||||
|
!p. 126) and Jdiff [mol ! |
s–1] by Vdiff |
[m3! s–1]. |
|||||||||||||
|
k · α · D is then summarized as diffusion con- |
|||||||||||||||
|
ductance, or Krogh’s diffusion coefficient K [m2 ! |
|||||||||||||||
|
s–1 ! Pa–1]. Substitution into Fick’s first diffusion |
|||||||||||||||
|
equation yields |
|
|
|
|
|
|
|
||||||||
|
. |
|
|
|
|
|
|
|
|
|
|
|
|
|
||
|
|
Vdiff |
|
! K ! |
|
P |
|
[m ! s–1]. |
[1.7] |
|||||||
|
|
A |
|
|
x |
|
||||||||||
|
|
|
|
|
|
|
|
|
|
|
|
|
|
|||
|
Since |
A and |
|
x of |
alveolar gas exchange |
|||||||||||
|
(!p. 120) cannot be determined in living or- |
|||||||||||||||
|
ganisms, K · F/ |
x for O2 is often expressed as |
||||||||||||||
|
the O2 |
diffusion capacity of the lung, DL: |
|
|||||||||||||
|
. |
|
|
|
|
|
PO2 [m3 ! s–1]. |
|
|
|||||||
|
|
VO2diff ! DL ! |
[1.8] |
|||||||||||||
|
Nonionic diffusion occurs when the uncharged |
|||||||||||||||
|
form of a weak base (e.g., ammonia = NH3) or |
|||||||||||||||
|
acid (e.g., formic acid, HCOOH) passes through |
|||||||||||||||
|
a membrane more readily than the charged |
|||||||||||||||
22 |
form (!F). In this case, the membrane would |
|||||||||||||||
be more permeable |
to |
NH3 than |
to NH4+ |
(!p. 176 ff.). Since the pH of a solution determines whether these substances will be charged or not (pK value; !p. 378), the diffusion of weak acids and bases is clearly dependent on the pH.
The previous equations have not made allowances for the diffusion of electrically charged particles (ions). In their case, the electrical potential difference at cell membranes must also be taken into account. The electrical potential difference can be a driving force of diffusion (electrodiffusion). In that case, positively charged ions (cations) will then migrate to the negatively charged side of the membrane, and negatively charged ions (anions) will migrate to the positively charged side. The prerequisite for this type of transport is, of course, that the membrane contain ion channels (!p. 32 ff.) that make it permeable to the transported ions. Inversely, every ion diffusing along a concentration gradient carries a charge and thus creates an electric diffusion potential (!p. 32 ff.).
As a result of the electrical charge of an ion, the permeability coefficient of the ion x (= Px) can be transformed into the electrical conductance of the membrane for this ion, gx (!p. 32):
gx ! ! Px ! zx2 ! F2 R–1 ! T–1 ! |
|
x [S ! m–2] |
[1.9] |
c |
where R and T have their usual meaning (explained above) and zx equals the charge of the ion, F equals the Faraday constant (9,65 ! 104 A ! s ! mol–1), and cx equals the mean ionic activity in the membrane. Furthermore,
c ! |
c1 |
–c2 . |
[1.10] |
|
lnc1 |
||||
|
– lnc2 |
|
where index 1 = one side and index 2 = the other side of the membrane. Unlike P, g is concentration-depend- ent. If, for example, the extracellular K+ concentration rises from 4 to 8 mmol/kg H2O (cytosolic concentration remains constant at 160 mmol/kg H2O), c will rise, and g will increase by 20%.
Since most of the biologically important substances are so polar or lipophobic (small kvalue) that simple diffusion of the substances through the membrane would proceed much too slowly, other membrane transport proteins called carriers or transporters exist in addition to ion channels. Carriers bind the target molecule (e.g., glucose) on one side of the membrane and detach from it on the other side
Despopoulos, Color Atlas of Physiology © 2003 Thieme
All rights reserved. Usage subject to terms and conditions of license.

D. Permeability of lipid membranes |
|
E. Facilitated diffusion |
|
||||||||
3•10–5 |
|
Methanol |
Triethyl |
|
|
|
|
II |
|||
|
|
|
|
|
|
citrate |
|
|
Facilitated diffusion |
Transport by Means of Diffusion |
|
) |
|
|
|
|
Ethanol |
Trimethyl citrate |
|
||||
|
|
|
|
|
(see G. for carriers) |
||||||
–1 |
|
|
|
|
|
|
|||||
|
|
|
|
|
Antipyrine |
|
|
||||
|
|
|
|
|
|
|
] |
|
|
||
|
|
|
|
|
|
Valeramide |
|
|
|
||
|
|
|
Cyanamide |
|
–1 |
|
n |
||||
|
|
–6 |
|
|
•s |
|
|||||
• |
|
|
|
|
|
Diacetin |
|
ti |
|
||
3 10 |
|
|
|
|
|
|
–2 |
a |
|
||
|
|
|
|
|
Butyramide |
|
r |
|
|||
|
|
|
Acetamide |
|
|
|
u |
|
|||
|
|
|
|
|
|
|
|
t |
|
||
|
|
|
|
Chlorohydrin |
|
|
a |
|
|||
|
|
|
Ethylene |
|
|
|
S |
|
|||
|
|
|
|
Succinamide |
|
|
|
|
|||
|
|
|
glycol |
|
Dimethylurea |
|
|
|
|
||
3•10–7 |
Methyl- |
|
Ethylurea |
|
|
|
|
|
|||
|
|
|
urea |
|
Diethylmalonamide |
|
|
|
|
||
|
|
|
Urea |
|
|
|
|
|
|||
|
|
|
|
|
|
|
|
|
|
||
3•10–8 |
|
|
|
(Sphere diameter |
|
Simple diffusion |
|||||
|
|
|
|
|
|
||||||
coefficientPermeability(m•s |
|
|
Glycerol |
|
= molecular radius) |
Transport[molratem• |
|
|
|||
|
|
|
|
|
Collander(Datafromal.)et |
|
|
||||
|
|
10–4 |
10–3 |
10–2 |
10–1 |
|
C[mol•m–3] |
||||
|
|
|
1 |
|
|
Passive |
|||||
|
|
|
Distribution coefficient k for olive oil/water |
|
|
|
|||||
F. Nonionic diffusion |
|
G. Passive carrier transport |
|||||||||
|
|
|
|
|
|
|
|
|
Carrier |
|
1.11 |
H+ |
+ NH4+ |
|
NH4+ + |
H+ |
|
|
protein |
|
|||
|
|
|
|
|
|
|
|
|
Plate |
||
|
|
|
|
|
|
|
|
|
|
|
|
|
|
|
NH3 |
|
NH3 |
|
|
|
|
|
|
|
|
|
|
|
|
|
|
|
|
|
|
H+ + HCOO– |
|
HCOO– + |
H+ |
|
|
|
|
|
|||
|
|
|
|
|
|
|
|
|
|
||
|
HCOOH |
|
|
|
|
|
|
|
|
||
|
|
|
|
|
HCOOH |
|
|
|
|
|
|
(after a conformational change) (!G). As in simple diffusion, a concentration gradient is necessary for such carrier-mediated transport (passive transport), e.g., with GLUT uniporters for glucose (!p. 158). On the other hand, this type of “facilitated diffusion” is subject to satu-
ration and is specific for structurally similar substances that may competitively inhibit one another. The carriers in both passive and active transport have the latter features in common (!p. 26).
23
Despopoulos, Color Atlas of Physiology © 2003 Thieme
All rights reserved. Usage subject to terms and conditions of license.

1 Fundamentals and Cell Physiology
24
Osmosis, Filtration and Convection
Water flow or volume flow (JV) across a membrane, in living organisms is achieved through osmosis (diffusion of water) or filtration. They can occur only if the membrane is water-per- meable. This allows osmotic and hydrostatic pressure differences (Δπ and P) across the membrane to drive the fluids through it.
Osmotic flow equals the hydraulic conductivity (Kf) times the osmotic pressure difference (Δπ) (!A):
JV ! Kf ! Δπ |
[1.11] |
The osmotic pressure difference (Δπ) can be calculated using van’t Hoff’s law, as modified by Staverman:
Δπ ! σ ! R ! T ! Cosm, |
[1.12] |
where σ is the reflection coefficient of the particles (see below), R is the universal gas constant (! p. 20), T is the absolute temperature,
and Cosm [osm ! kgH2O–1] is the difference between the lower and higher particle concen-
trations, Caosm –Cbosm (!A). Since Cosm, the driving force for osmosis, is a negative value, JV
is also negative (Eq. 1.11). The water therefore flows against the concentration gradient of the solute particles. In other words, the higher concentration, Cbosm, attracts the water. When the concentration of water is considered in osmosis, the H2O concentration in A,a, CaH2O, is greater than that in A,b, CbH2O. CaH2O –CbH2O is therefore the driving force for H2O diffusion
(!A). Osmosis also cannot occur unless the reflection coefficient is greater than zero (σ "0), that is, unless the membrane is less permeable to the solutes than to water.
Aquaporins (AQP) are water channels that permit the passage of water in many cell membranes. A chief cell in the renal collecting duct contains a total of ca. 107 water channels, comprising AQP2 (regulated) in the luminal membrane, and AQP3 and 4 (permanent?) in the basolateral membrane. The permeability of the epithelium of the renal collecting duct to water (!A, right panel) is controlled by the insertion and removal of AQP2, which is stored in the membrane of intracellular vesicles. In the presence of the antidiuretic hormone ADH (V2 receptors, cAMP; ! p. 274), water channels are inserted in the luminal membrane within minutes, thereby increasing the water permeability of the membrane to around 1.5 #10– 17 L s– 1 per channel.
In filtration (!B), |
|
JV ! Kf ! P – Δπ |
[1.13] |
Filtration occurs through capillary walls, which allow the passage of small ions and molecules (σ = 0; see below), but not of plasma proteins (!B, molecule x). Their concentration difference leads to an oncotic pressure difference (Δπ) that opposes P. Therefore, filtration can occur only if P "Δπ (!B, p. 152, p. 208).
Solvent drag occurs when solute particles are carried along with the water flow of osmosis or filtration. The amount of solvent drag for solute X (JX) depends mainly on osmotic flow (JV) and the mean solute activity ax (! p. 376) at the site of penetration, but also on the degree of particle reflection from the membrane, which is described using the reflection coefficient (σ). Solvent drag for solute X (JX) is therefore calculated as
Jx ! JV (1 – σ) ax [mol ! s–1] [1.14] Larger molecules such as proteins are entirely
reflected, and σ = 1 (!B, molecule X). Reflection of smaller molecules is lower, and σ$ 1. When urea passes through the wall of the proximal renal tubule, for example, σ = 0.68. The value (1–σ) is also called the sieving coefficient (! p. 154).
Plasma protein binding occurs when smallmolecular substances in plasma bind to proteins (!C). This hinders the free penetration of the substances through the endothelium or the glomerular filter (! p. 154 ff.). At a glomerular filtration fraction of 20%, 20% of a freely filterable substance is filtered out. If, however, 9/10 of the substance is bound to plasma proteins, only 2% will be filtered during each renal pass.
Convection functions to transport solutes over long distances—e.g., in the circulation or urinary tract. The solute is then carried along like a piece of driftwood. The quantity of solute
transported over time (Jconv) is the product of volume flow JV (in m3 ! s–1) and the solute con-
centration C (mol ! m–3):
Jconv ! JV ! C [mol ! s–1]. [1.15] The flow of gases in the respiratory tract, the transmission of heat in the blood and the release of heat in the form of warmed air occurs through convection (! p. 222).
Despopoulos, Color Atlas of Physiology © 2003 Thieme
All rights reserved. Usage subject to terms and conditions of license.

A. Osmosis (water diffusion) |
|
|
|
|
|
|
|
Caosm |
π |
Cbosm |
|
b |
Example |
Lumen |
Interstice |
|
C |
||||||
CaH2O |
|
|
H2O |
|
|
|
|
|
|
|
|
H2O |
|
||
|
|
|
|
b |
a |
Aqua- |
|
|
|
|
|
C osm > C osm, |
porins |
|
|
|
|
|
|
|
i.e., |
|
|
|
|
|
|
|
|
|
|
H2O |
|
|
|
CaH2O > CbH2O |
|
|
|
a |
|
b |
|
Water diffusion |
|
|
|
|
|
|
|
from a to b |
|
|
|
|
|
|
|
|
|
|
Epithelium |
|
|
|
|
|
|
|
of renal |
|
Water flux JV = Kf · |
π (~ Caosm– Cbosm) |
collecting duct |
||||
|
|
|
B. Filtration
|
|
|
P |
|
|
|
|
|
||||||
Pa |
Example |
|
|
|
|
|
||||||||
|
|
|
|
|
|
Pb |
|
|
|
Glomerular |
|
|
||
|
|
|
|
|
|
|
|
|
|
|
capillary |
|
|
|
|
|
|
|
|
|
|
|
|
|
|
|
|
||
|
|
|
|
|
|
|
|
|
|
|
|
|
|
|
|
|
|
|
|
|
|
|
|
|
|
|
|
|
|
|
|
|
|
|
|
|
|
Pa > Pb |
|
|
|
|
||
|
|
|
|
|
|
|
|
and |
πx |
|
|
|
|
|
|
|
|
|
|
|
|
|
|
|
|
|
|||
a |
x |
|
|
|
|
P > |
|
Blood |
|
|
||||
|
|
|
|
|
|
|
||||||||
|
|
|
|
|
|
|
|
|
|
|||||
|
|
|
|
|
|
|
|
|
||||||
|
|
|
|
|
|
b |
|
|
|
|
|
|
||
|
|
|
|
|
|
|
|
Water filtration |
|
|
|
|
||
|
|
|
|
|
|
|
|
from a to b |
|
|
|
|
||
|
|
|
|
|
|
|
|
|
|
|
|
|||
|
|
|
|
|
|
|
|
|
|
|
|
|||
|
|
|
πx |
|
|
πx) |
|
|
P |
|
|
π |
||
|
Water flux JV = Kf · ( P– |
|
Primary |
Filtrate |
|
|||||||||
|
|
|
(= oncotic pressure |
|||||||||||
|
|
|
|
|
|
|
|
|
|
urine |
|
|
of plasma proteins) |
|
|
|
|
|
|
|
|
|
|
|
|
|
|
|
C. Plasma protein binding
Protein |
Blood |
|
side |
||
|
a
H2O
Prevents excretion
(e.g., binding of heme by hemopexin)
Transports substances in blood
(e.g., binding of Fe3+ ions by apotransferrin)
Provides rapid access ion stores (e.g., of Ca2+ or Mg2+)
Helps to dissolve lipophilic substances in blood (e.g., unconjugated bilirubin)
bAffects certain medications (e.g., many sulfonamides): Protein-bound fraction
–not pharmacologically active
–not filtratable (delays renal excretion)
–functions as an allergen (hapten)
Plate 1.12 Osmosis, Filtration and Convection
25
Despopoulos, Color Atlas of Physiology © 2003 Thieme
All rights reserved. Usage subject to terms and conditions of license.

1 Fundamentals and Cell Physiology
26
Active Transport |
and, thus, for maintenance of the cell mem- |
||||
brane potential. During each transport cycle |
|||||
|
|||||
Active transport occurs in many parts of the |
(!A1, A2), 3 Na+ and 2 K+ are “pumped” out of |
||||
body when solutes are transported against |
and into the cell, respectively, while 1 ATP |
||||
their concentration gradient (uphill transport) |
molecule is used to phosphorylate the carrier |
||||
and/or, in the case of ions, against an electrical |
protein (!A2b). Phosphorylation first |
||||
potential (! p. 22). All in all, active transport |
changes the conformation of the protein and |
||||
occurs against the electrochemical gradient or |
subsequently alters the affinities of the Na+ |
||||
potential of the solute. Since passive transport |
and K+ binding sites. The conformational |
||||
mechanisms represent “downhill” transport |
change is the actual ion transport step since it |
||||
(! p. 20 ff.), they are not appropriate for this |
moves the binding sites to the opposite side of |
||||
task. Active transport requires the expenditure |
the membrane (!A2b – d). Dephosphoryla- |
||||
of energy. A large portion of chemical energy |
tion restores the pump to its original state |
||||
provided by foodstuffs is utilized for active |
(!A2e – f). The Na+/K+ pumping rate increases |
||||
transport once it has been made readily avail- |
when the cytosolic Na+ concentration rises— |
||||
able in the form of ATP (! p. 41). The energy |
due, for instance, to increased Na+ influx, or |
||||
created by ATP hydrolysis is used to drive the |
when the extracellular K+ rises. Therefore, |
||||
transmembrane transport of numerous ions, |
Na+,K+-activatable ATPase is the full name of |
||||
metabolites, and waste products. According to |
the pump. Na-+K+-ATPase |
is inhibited by |
|||
the laws of thermodynamics, the energy ex- |
ouabain and cardiac glycosides. |
|
|||
pended in these reactions produces order in |
Secondary active transport occurs when |
||||
cells and organelles—a prerequisite for sur- |
uphill transport of a compound (e.g., glucose) |
||||
vival and normal function of cells and, there- |
via a carrier protein (e.g., sodium glucose |
||||
fore, for the whole organism (! p. 38 ff.). |
transporter type 2, SGLT2) is coupled with the |
||||
In primary active transport, the energy pro- |
passive (downhill) transport of an ion (in this |
||||
duced by hydrolysis of ATP goes directly into |
example Na+; !B1). In this case, the electro- |
||||
ion transport through an ion pump. This type |
chemical Na+ gradient into the cell (created by |
||||
of ion pump is called an ATPase. They establish |
Na+-K+-ATPase at another site on the cell mem- |
||||
the electrochemical gradients rather slowly, |
brane; !A) provides the driving force needed |
||||
e.g., at a rate of around 1 µmol ! s–1 ! m–2 of |
for secondary active uptake of glucose into the |
||||
membrane surface area in the case of Na+-K+- |
cell. Coupling of the transport of two com- |
||||
ATPase. The gradient can be exploited to |
pounds across a membrane is called cotrans- |
||||
achieve rapid ionic currents in the opposite |
port, which may be in the form of symport or |
||||
direction after the permeability of ion chan- |
antiport. Symport occurs when the two com- |
||||
nels has been increased (! p. 32 ff.). Na+ can, |
pounds (i.e., compound and driving ion) are |
||||
for example, be driven into a nerve cell at a rate |
transported across the membrane in the same |
||||
of up to 1000 µmol ! s–1 ! m–2 during an action |
direction (!B1–3). Antiport (countertrans- |
||||
potential. |
port) occurs when they are transported in op- |
||||
ATPases occur ubiquitously in cell mem- |
posite directions. Antiport occurs, for example, |
||||
branes (Na+-K+-ATPase) and in the endo- |
when an electrochemical Na+ gradient drives |
||||
plasmic reticulum and plasma membrane |
H+ in the opposite direction by secondary ac- |
||||
(Ca2+-ATPase), renal collecting duct and stom- |
tive transport (!B4). The resulting H+ gradient |
||||
ach glands (H+,K+ -ATPase), and in lysosomes |
can then be exploited for tertiary active sym- |
||||
(H+-ATPase). They transport Na+, K+, Ca2+ and |
port of molecules such as peptides (!B5). |
||||
H+, respectively, by primarily active mecha- |
Electroneutral transport occurs when the |
||||
nisms. All except H+-ATPase consist of 2 α-sub- |
net electrical charge remains balanced during |
||||
units and 2 "-subunits (P-type ATPases). The |
transport, e.g., during Na+/H+ antiport (!B4) |
||||
α-subunits are phosphorylated and form the |
and Na+-Cl– symport (!B2). Small charge sep- |
||||
ion transport channel (!A1). |
aration occurs in electrogenic (rheogenic) |
||||
Na+-K+-ATPase is responsible for main- |
transport, e.g., in |
Na+-glucose0 |
symport |
||
tenance of intracellular Na+ and K+ homeostasis |
(!B1), Na+-amino |
acid0 |
symport |
(!B3), |
!
Despopoulos, Color Atlas of Physiology © 2003 Thieme
All rights reserved. Usage subject to terms and conditions of license.

+ |
+ |
|
|
|
|
|
A. Na , K - ATPase |
|
|
|
|||
|
|
|
3 Na+ |
|
|
|
|
1 |
Outside |
[Na+]o |
|
[K+]o |
|
|
|
|
|
|||
|
|
β |
β |
|
|
|
|
|
|
|
|
||
|
|
membrane |
α |
α |
|
|
|
|
Cytosol Cell |
[Na+]i |
ADP |
[K+]i |
Active Transport I |
|
|
|
ATP |
|
||
|
|
|
2 K+ |
|
||
2 |
|
a |
|
b |
|
1.13 |
|
|
|
|
Plate |
||
|
|
|
|
|
|
|
|
|
|
|
|
P |
|
|
|
|
|
ATP |
|
|
|
|
|
Na+ binding, |
Phosphorylation |
Confor- |
|
|
|
|
K+ discharge |
|
High affinity |
|
|
|
|
|
|
mational |
|
|
|
|
|
|
change |
for K+ |
|
|
|
|
|
c |
|
f |
|
|
Conformation E1 |
|
|
|
|
|
|
|
|
|
|
|
|
|
|
|
|
Na+ |
|
|
K+ |
|
|
|
P |
|
|
|
|
|
|
|
hochaffin |
Conformation E2 |
|
|
|||
|
d |
|
||||
Na+ |
|
|
|
|
||
Conformational |
|
e |
|
|
||
change |
|
|
|
|
|
|
|
|
|
|
|
|
|
|
|
|
|
|
|
P |
|
|
|
|
|
Na+ discharge, |
|
|
|
|
|
Pi |
K+ binding |
27 |
|
|
|
Dephosphorylation |
|
||
|
|
|
(Partly after P. Läuger) |
Despopoulos, Color Atlas of Physiology © 2003 Thieme
All rights reserved. Usage subject to terms and conditions of license.