
Biomedical EPR Part-B Methodology Instrumentation and Dynamics - Sandra R. Eaton
.pdf312 |
DEREK MARSH ET AL. |
to biomedical applications. The result – the second-harmonic 90°-out-of- phase absorption display, – was the next and lasting breakthrough in the development of ST-EPR. Indeed, this is the non-linear display with which saturation transfer spectroscopy has come to be identified. Apart from the adequate intensity of the
its success can be attributed to the richness of the lineshapes. Qualitatively, the
appears as an admixture of the out-of-phase first-harmonic dispersion
with the in-phase second-harmonic absorption
A comprehensive experimental analysis, including simulations, was presented of the dependence of the (and
) ST-EPR spectra on the rotational correlation time of spin-labelled haemoglobin in the publication by Thomas, Dalton and Hyde (1976). This was an enormously influential paper and remains the classic reference. Sensitivity of the
to rotational correlation time was demonstrated over almost four decades from
to
Qualitatively, the proportion of
contribution to the
decreases with decreasing correlation time. For shorter correlation times, contributions from incipient motional narrowing of the
component contribute also to the motional sensitivity of the
The paper by Thomas, Dalton and Hyde (1976) on spin-labelled haemoglobin represents the first detailed application of ST-EPR to a biomolecular system. It also established the most practical method for analysis of the correlation-time dependence of the
lineshapes in terms of the diagnostic lineheight ratios L"/L, C'/C and H"/H. These represent the ratios of the
L", C' and H" in the spectral regions of maximum angular dispersion to those L, C and H at the stationary angular turning points, for the low-field, central and high-field manifolds, respectively. Already, the utility of these lineheight ratios had been anticipated in ST-EPR simulations by Thomas and McConnell (1974) using the diffusion-coupled Bloch equations. Together with elaborations, e.g., for the greater angular dispersion in high-field spectra (Johnson and Hyde, 1981), this remains the most useful empirical approach to analysis of STEPR lineshapes.
The initial work on spin-labelled haemoglobin (Hyde and Thomas, 1973) was quickly followed by the application to muscle proteins by Thomas, Hyde, Seidel and Gergely (1975). This represents the first truly biomedical application of ST-EPR and firmly establishes the utility of the method for the study of supramolecular aggregates. The application to biological membranes came somewhat later. Rhodopsin was the first membrane protein to be studied by ST-EPR (Baroin et al., 1977). This again was a landmark study because it represents the other major biological ST-EPR application, in addition to supramolecular assemblies, viz., to the rotational diffusion of integral proteins in the high-viscosity lipid environment of membranes (see
SATURATION TRANSFER SPECTROSCOPY |
313 |
also Hidalgo et al., 1978; Kirino et al., 1978; Kusumi et al., 1978; Rousselet and Devaux, 1977). A study of the rotational mobility of spin-labelled lipids in gel-phase membranes (Marsh, 1980) was the first to consider the effects of rotational anisotropy on ST-EPR spectra. These and other earlier applications were reviewed by Hyde and Dalton (1979). An early exposition of the methodology is by Hyde (1978).
In principle, the basic foundations of all further developments in saturation transfer spectroscopy were set by the Hyde and Thomas (1973), and the Thomas, Dalton and Hyde (1976) papers. Robinson and Dalton contributed considerably to the development of theoretical simulations, particularly with respect to anisotropic rotational diffusion (Robinson and Dalton, 1980). Evans (1981) was the first to suggest correlation-time analysis by using the integrated intensity of the spectrum. The purpose of this was to remove the effects of contaminating fast tumbling spin labels which contribute little to the integrated ST-EPR intensity but disturb the measurement of lineheight ratios. It was later recognised that this is a viable method for analysing general two-component ST-EPR spectra (Horváth and Marsh, 1983). Use of ST-EPR integrals then led to the discovery of the sensitivity to weak spin-spin interactions (Horváth et al., 1990) and ultimately to establishing out-of-phase intensities as a method to determine relaxation enhancements (Marsh et al., 1998). The final step in this development is the demonstration of the first harmonic out-of-phase absorption
spectrum as a “pure”
display (Livshits et al., 1998a), as proposed originally by Hyde and Thomas (Hyde and Thomas, 1973).
3.RAPID-PASSAGE SATURATION-TRANSFER- EPR DISPLAYS
The fundamental basis of saturation transfer spectroscopy is to use nonlinear CW detection under conditions of partial microwave saturation. In rapid passage experiments, this is invariably done by detecting in phase quadrature with the Zeeman field modulation. However, as a CW technique, progressive saturation also belongs generically to the nonlinear class of experiments. The second aspect of saturation transfer spectroscopy is spectral resolution: saturation is transferred from one spin packet to a spin packet elsewhere in the spectrum. The rate of transfer must be comparable to the spin-lattice relaxation rate. Spectral intensities are then a measure of the rate of the process that causes the transfer of saturation. Orientational selection of spin packets gives rise to sensitivity of powder lineshapes to slow rotational diffusion. This is the classical saturation
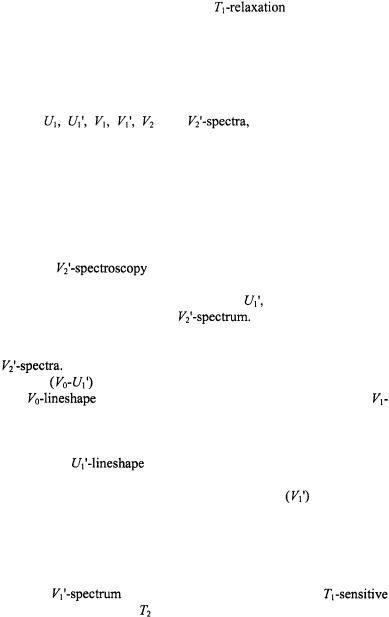
314 |
DEREK MARSH ET AL. |
transfer experiment. On the other hand, |
enhancement by |
paramagnetic relaxants cause a decrease in intensity with little effect on lineshape. This is the non-classical saturation transfer experiment that provides certain advantages over progressive saturation experiments.
Out-of-phase detected EPR spectra that have been investigated are the first-harmonic dispersion signal and absorption signals up to the second
harmonic |
(Hyde and Thomas, |
1973). With the notation already introduced |
|
these are |
the |
and |
respectively, where the |
prime indicates out-of-phase detection. Fig. 2 gives simulated spectra illustrating these six displays. Of the nonlinear (i.e., out-of-phase) displays, the first harmonic dispersion and second-harmonic absorption are sensitive to ultraslow rotational diffusion. This was demonstrated experimentally by Hyde and Thomas (1973) with both the small spin label hydroxy-TEMPO in supercooled sec-butyl benzene and maleimide spin-labelled haemoglobin in glycerol. They found that the second harmonic absorption out-of-phase display has the greatest sensitivity of lineshape to rotational correlation time.
For this reason, |
has become the standard method to study |
|
very slow rotation of spin-labelled biomolecular assemblies. |
||
In principle, the first harmonic dispersion, |
out-of-phase display |
|
contains the same information as the |
However, the lineshape |
changes induced by saturation transfer are not so richly detailed and do not lend themselves so easily to the definition of diagnostic lineheight ratios as
do the |
Fajer and Marsh (1983a) introduced a method of analysis |
||
based on the |
difference |
spectrum, where the |
zeroth-harmonic |
absorption |
is obtained |
by integration of the |
unsaturated |
Rotational correlation time calibrations were produced for
by using spin-labelled haemoglobin. This offers a general approach to detecting saturation transfer even in complex or multicomponent systems.
Deviations of the |
from that of the conventional integrated |
|
absorption spectrum are a quantitative indication of saturation transfer. |
||
The first-harmonic |
out-of-phase absorption spectrum |
is insensitive |
to rotational motion (see Fig. 2). Hyde and Thomas (1973) suggested, however, that this nonlinear rapid passage display might be useful for obtaining information on spin-lattice relaxation times. Indeed, this has formed the basis for development of the non-classical type of saturation transfer experiment that was referred to above. As will be seen later in
section 7.2, the |
turns out to be practically an ideal |
|
display, with little sensitivity to |
or molecular motion. |

SATURATION TRANSFER SPECTROSCOPY |
315 |
Figure 2. Simulated out-of-phase (right-hand side) and in-phase (left-hand side) nitroxide EPR spectra. Top row: first harmonic dispersion, and
middle row: first harmonic absorption,
and
bottom row: second harmonic absorption,
and
Spectra are simulated for spin-label rotation rates of
(solid line) and
(dashed line). The simulation procedure is described later in Section 4.
4.MODULATION-COUPLED BLOCH EQUATIONS
Simulation of rapid-passage nonlinear EPR spectra requires explicit inclusion not only of the microwave magnetic field, but also of the Zeeman modulation field. Halbach (1954) provided analytical solutions of the Bloch equations for the first harmonic out-of-phase absorption and dispersion signals, in the low amplitude limit. This was subsequently extended to the second harmonic absorption signal, for studying the dependence of the out- of-phase signal on time (Páli et al., 1996). Analysis of slow molecular motion by ST-EPR necessitates use of the diffusion-coupled Bloch equations. This was first done by Thomas and McConnell (1974) with a Brownian diffusion model, and used to validate rotational correlation time calibrations based on diagnostic lineheight ratios (Thomas et al., 1976). Solutions must be obtained numerically when dealing with small-step


SATURATION TRANSFER SPECTROSCOPY |
317 |
is obtained from expansion of the Bloch equation matrix, and the gyromagnetic ratio tensor is:
Here is the resonance field:
with the microwave frequency and
the nuclear magnetic quantum number of the spin label. The
and hyperfine tensor anisotropies are given by the intermediate field approximation (Van et al., 1974):
where the principal tensor components are: and
Pseudo-secular terms are retained in Eqs. 7,8, and line positions are well reproduced, although non-secular ocuplings are neglected.
A solution is obtained in the small modulation amplitude approximation by expanding the Fourier coefficients in a power series
of the dimensionless modulation amplitude, (Halbach, 1954):



320 |
DEREK MARSH ET AL. |
5.SLOW ROTATIONAL DIFFUSION
The sensitivity of nonlinear ST-EPR spectra to slow rotational motion can be analysed directly by spectral simulation using the diffusion-coupled Bloch equations, as already described and illustrated in Fig. 3. However, an approximate semi-analytical approach based on the formal equivalence between Heisenberg spin exchange and exchange by jump diffusion (Eastman et al., 1969; Marsh, 1992a) has the advantage of containing the basic physical principles of saturation transfer and of giving rise to a very simple expression for parameterising experimental correlation time calibrations (Marsh and Horváth, 1992a). For these reasons, particularly the
latter, we outline this simplified treatment here. |
|
|
|
The effective spin-lattice relaxation time, |
for a given |
spin |
|
packet at resonance position |
depends on the |
spectral diffusion |
rate, |
according to (Eastman et al., 1969; Marsh, 1992a) (and see later in Section 8.1.2):
where is the intrinsic spin-lattice relaxation time (in the absence of spectral diffusion) and
is the fractional spin-packet population, or degeneracy (see Section 8.1.2). Thus,
is the redistribution factor of the spin packet at position
on spectral diffusion over the entire powder lineshape. The spectral diffusion rate at resonance position
is given by (Fajer et al., 1986):
where is the rotational correlation time and
is the spin packet width
by which the resonance position must change in order to alleviate saturation |
||
(cf. Fig. 1). This expression (i.e., Eq. 18) was used by Fajer, Hyde and |
||
coworkers (1986) to analyse the fast phase in the saturation recovery of spin- |
||
labelled haemoglobin, |
following |
short microwave pulses. The parameter |
is the rate at |
which the |
resonance position changes with angular |
orientation, of the spin label with respect to the magnetic field direction (see Hyde and Dalton, 1979).
As will be seen later (in section 7.3), the intensity of the out-of-phase STEPR signal, relative to the conventional in-phase EPR, is approximately proportional to (Páli et al., 1996). The ST-EPR intensity can therefore

SATURATION TRANSFER SPECTROSCOPY |
321 |
be approximated by: where
is the intensity in the absence of spectral diffusion (Marsh and Horváth, 1992b). Hence from Eq. 17, the ST-EPR lineshape is given by:
where the degeneracy factor or fractional population, for a spectral segment of width
is given by
The following axial orientation dependence of the resonance position contains the essential features of anisotropic powder patterns (cf. Marsh, 1990):
where are the resonance line positions corresponding to the magnetic field oriented parallel or perpendicular to the principal axis, i.e.,
and
respectively. The resulting rate of change of the resonance position with angle is:
and the normalised lineshape in the absence of rotational diffusion is:
which is valid for the range
Figure 4 gives the model lineshapes predicted from Eqs. 18-22 for various values of the rotational correlation time, The calculations reproduce the well-known sensitivity of ST-EPR lineshapes to rotational diffusion at rates comparable to that of the spin-lattice relaxation, which was characterised originally by Thomas, Hyde and coworkers (1976). The lineheight at an intermediate spectral position P', relative to that at the invariant turning point P, decreases progressively with decreasing correlation time. The inset to Fig. 4 demonstrates that the relative intensity at a position 1/3 of the way in from the
turning point depends on rotational correlation time in a manner very similar to that found for the experimental diagnostic ST-EPR lineheight ratios L"/L, C'/C and H"/H (see Thomas et al., 1976).