
Biomedical EPR Part-B Methodology Instrumentation and Dynamics - Sandra R. Eaton
.pdf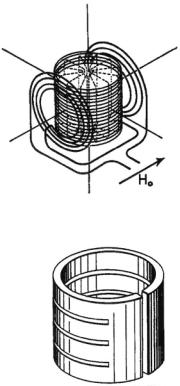
414 |
JAMES S. HYDE |
approaches can also affect the microwave fields, and that the final design will require iteration between both software packages.
Figure 1. Schematic diagram of wire-wound cavity and field modulation coils.
Figure 2. Outline drawing of the one-loop—one-gap resonator used for multifrequency EPR in the frequency range of 0.5 to 8 GHz. Hyde and Froncisz (1989) contains additional detail. The horizontal slots permit penetration of the 100 kHz magnetic field modulation.
There are several consequences of these advances in simulation of electromagnetic microwave and modulation fields. i) Design efficiency is greatly improved, which facilitates development of resonators that are optimized for specific applications. This, in turn, leads to an increase in sensitivity for the particular application. Previously, a resonator that was suitable for a broad class of applications was generally employed, which often resulted in a compromise with respect to sensitivity. ii) Cavity coupling structures, sample access stacks, dewar inserts and sample geometries can now be designed on a rational basis rather than the highly intuitive basis of the past. iii) A proliferation of resonators for use at frequencies above and below X-band can be expected that are modeled after the rich array of X-band designs.
TRENDS IN EPR TECHNOLOGY |
415 |
It is concluded from this experience that the software and computing capability has reached the point where optimization of design is better carried out by computer than by experiment. Lower symmetry structures with a large number of design parameters can be investigated by computer modeling that would be impractical to investigate by construction of a series of actual cavities that could be experimentally probed to explore this parameter space.
The conclusion of this section is that we are truly on the verge of a paradigm shift in EPR resonator design that will enable optimization of the structure for any set of sample constraints. The new modeling tools fundamentally increase efficiency in resonator design. One can speculate that commercial design of resonators optimized for specific problems and sold in small numbers may become economically feasible. And there may well be other novel structures waiting to be discovered, in analogy to the discovery of UF modes.
3.NOISE
In the previous section, we discussed optimization of microwave resonator design to maximize the EPR signal. Now we discuss minimizing noise. Together, the goal is to identify future opportunities to improve the signal-to-noise ratio (SNR).
In continuous wave EPR, noise originating in the microwave oscillator and noise originating in the detection system as characterized by the overall receiver noise figure are of primary concern. At high microwave powers incident on the EPR resonator, noise from the oscillator dominates, while at low incident powers, receiver noise dominates. We have found that plots of spectrometer noise versus incident power give considerable insight into spectrometer performance. Six such plots in log-log format are shown in Figs. 3-5. They have been redrawn for display consistency from figures in (Hyde et al., 1982; Froncisz et al., 1986; Hyde et al., 1991; Lesniewski and Hyde, 1990; Pfenninger et al., 1995). Figures 3 and 5 are plots of noise voltage and Fig. 4 is of noise power. Note the differences in scales of the ordinate and abscissa comparing Figs. 3 and 5 with Fig. 4. At low incident powers, noise is independent of power, while at high incident power, the noise voltage varies linearly with incident power. The intercept of these two dependencies results in a “breakpoint” where the noise from each source is the same.
Figure 3a was taken from (Hyde et al., 1982), which was concerned with phase noise 100 kHz from the carrier frequency of the oscillator when tuned to the dispersion. The overall receiver noise figure that limits spectrometer
416 |
JAMES S. HYDE |
performance at low powers in Fig. 3a is estimated to be 11 dB. Comparison was made between a loop gap resonator with an unloaded Q-value of 650 and a standard Varian multipurpose rectangular cavity with an unloaded Q-value of 7900. Demodulation of phase noise depends on the resonator Q. One expects the ratio of break-point powers to be given by the square of the ratio of Qs, i.e., 22 dB, compared with the measured value of 25 dB, which was considered to be fair agreement. Not only is phase noise less troublesome when using low Q resonators, but the energy density in the LGR employed in this study was 65 times higher than in the cavity. It follows that studies in the dispersion mode can be carried out with the loop gap resonator over a much greater range of energy densities at the sample without encountering source phase noise – about 43 dB.
Figure 3b is similar to 3a, but displays data taken from Froncisz et al. (1986), which was a comparison at Q-band (35 GHz) of phase noise when using a cylindrical cavity resonator versus a two-loop—one-gap resonator. Comparison of Figs. 3a and 3b suggests the presence of substantially greater receiver noise at Q-band than X-band. The breakpoints occur at higher incident powers even though one would expect about 10 dB more phase noise from the oscillator at Q-band than at X-band (Robins, 1982). This can only occur if the receiver noise figure is substantially higher at Q-band than at X-band.
In order to improve EPR performance at Q-band, a low phase noise Gunn diode oscillator was developed in the author’s laboratory by R.A. Strangeway (Strangeway et al., 1995). A phase noise level in the range of -132 to -125 dBc/Hz at 100 kHz offset from the central frequency was achieved. This range can be compared with a measured value of -103 of the specific klystron in our Varian Q-band bridge (which was 4 dB worse than the manufacturer’s specification for a new klystron) as well as published values from the literature that fall in the range of -70 to -115. The -115 value was also from a cavity-stabilized Gunn oscillator. See Lesniewski and Hyde (1990) for additional detail. The phase noise that was achieved in Strangeway’s design remains, to the best of our knowledge, the lowest that has been reported at Q-band.
It is apparent from Figs. 3-5 that oscillator phase noise will become more evident if the overall receiver noise figure is reduced. Improvement in spectrometer performance over the widest range of conditions requires simultaneous reduction in receiver and oscillator noise. This concept was developed in detail in Hyde et al. (1991) and key results are displayed in Fig. 4. In that study, several changes were progressively made in the Q-band microwave bridge configuration, and noise was measured in each configuration as a function of incident microwave power while tuned to the dispersion using a cylindrical cavity resonator.

418 JAMES S. HYDE
In Fig. 4a, phase noise levels at the microwave bridge output utilizing a klystron and the low phase noise Gunn diode oscillator are compared. Breakpoints differed by 22 dB, which was in fair agreement with the value of 26 dB that was carefully measured on a microwave test bench. The data for this figure were obtained using a 1N53D point contact detector diode. The Gunn oscillator experiment of Fig. 4a was repeated using a more modern balanced mixer detector, and the results are shown in the lower graph of Fig. 4b. (Note that the results of Fig. 4a and 4b are not directly comparable because the gain of the preamplifier following microwave detection was reduced in the experiment of Fig. 4b.) A low noise microwave amplifier (LNA) with a specified noise figure of 4.3 dB and a gain of 25.5 dB was then inserted into the microwave bridge. Results are shown in the upper graph of Fig. 4b.
Notably, at low power the noise increased by only 14 dB, although the gain increased by 25.5 dB. A value for the noise figure of the balanced mixer 100 kHz from the carrier is readily calculated: 25.5 – 14 + 4.3 = 15.8 dB. This is not a very good number relative to X-band standards, but it was a considerable improvement relative to the 1N53D. At high powers, the two graphs in Fig. 4b are displaced by 25.5 dB, the gain of the LNA, as expected. The breakpoints are separated by 13 dB, in good agreement with the low power separation of 14 dB, demonstrating consistency of the data.
Figure 5a is derived from Lesniewski and Hyde (1990). It shows data at 19 GHz using a microwave bridge with a varactor-tuned Gunn oscillator. An AFC circuit was developed where the feedback loop had high gain at 100 kHz from the carrier. This enabled reduction of phase noise by 23 dB. This is viewed as an interesting and relatively unexplored strategy for reduction of microwave phase noise.
Pfenninger et al. (1995) reports data obtained at X-band using a cryogenic microwave preamplifier cooled to the temperature of liquid nitrogen with a 0.5 dB noise figure corresponding to 35 K equivalent noise temperature. As expected, phase noise originating from the microwave oscillator becomes more evident as shown in Fig. 5b. Data in this figure were obtained using a loop gap resonator observing either the dispersion or the absorption. The cryogenic amplifier had 15 db higher gain and 3 dB lower noise figure than the room temperature LNA with which it was compared, resulting in about 12 dB separation of the noise levels, as observed. The separation of the breakpoints for dispersion and absorption was about 35 dB. This value is dependent on the resonator Q, reference arm power, amplifier gains, and other details of the bridge setup and is not necessarily the same for the two amplifiers. Excess oscillator noise was clearly observable in the absorption mode configuration when using the cryogenic amplifier, and it would have been more apparent if a high Q cavity
TRENDS IN EPR TECHNOLOGY |
419 |
resonator had been utilized. Detailed analysis presented in this paper as well as in Rinard et al. (1999) predicted further improvement if the microwave circuit elements in the bridge were to be cooled. This paper is cast not only in the context of CW EPR, but also pulse EPR.
From the perspective of “Future Trends,” modern LNAs operating at room temperature seem adequate. Cooling of the amplifier combined with cooling of microwave bridge components may be desirable for selected experiments, but seems unlikely to become the general practice. However, improvement in microwave oscillator phase noise in CW EPR is feasible and desirable for two reasons: i) With an LNA in place, simultaneous detection of dispersion and absorption followed by a Hilbert transform of the dispersion and then addition of the two signals results in a highly desirable 3 dB improvement in SNR. Inspection of Figs. 3-5 indicates that phase noise remains excessive under practical conditions. Further reduction in oscillator phase noise is required in order to attain this improvement at higher levels of incident power. ii) As seen from the discussion concerning resonators, high Q cavities yield optimum performance for many classes of samples. In this case, reduced phase noise will be of value both for dispersion and absorption.
The Gunn diode itself is inherently noisy: the noise figure is on the order of 25 dB. Modern microwave transistors that could be used in an oscillator circuit have a noise figure on the order of a few dB. Continued improvements in transistor-based microwave oscillators have led to the recent development of low noise Yttrium-Iron-Garnet (YIG) oscillators. For example, standard manufactured YIG oscillators with a ±1 GHz electronic tuning range, 14 dBm power output and a center frequency anywhere in the 3 - 1 0 GHz range have a typical phase noise of -125 dBc/Hz at 100 kHz offset (Korber et al., 2002). Further improvement in future years can be anticipated.
Microwave frequency translation is employed in an increasing number of modern EPR microwave bridges. Examples include multiquantum EPR (Hyde, 1998a), time-locked sampling (ch. 7 in this volume), and translation of complex irradiation patterns formed at X-band to Q-band and also W- band for sample irradiation and then back to X-band for detection as employed in current Bruker spectrometer systems. Frequency translation can introduce additional sources of noise. The appendix of Strangeway et al. (1995) discusses several different approaches to frequency translation. One is to mix the output of a microwave oscillator with the output of a frequency synthesizer. Noise aspects of frequency synthesizers in the context of EPR spectroscopy are discussed in ch 7 of this volume.
As noise originating in the EPR spectrometer decreases, it is possible that noise originating in the environment will become dominant. Environmental
420 JAMES S. HYDE
noise includes microphonics in the audio frequency range and below, line frequency noise, instabilities in connections to ground, radio frequency interference, and temperature instabilities. The author has encountered each of these. It seems likely that future EPR spectrometer installations will be in shielded rooms with independent connections to ground, much as in magnetic resonance imaging installations. Mass storage devices, for example RAID arrays, permit storage of raw data with no signal averaging or filtering. This will allow inspection of the data for spurious environmental noise. It will also permit development of optimum filters of environmental noise.
EPR spectra are often obtained as a function of one or more additional experimental parameters, for example, temperature or time. Almost always in modern spectrometers the information is available in digital format, permitting the use of a variety of digital filters. Ideally, such filtering should be performed in multidimensional parameter space making use of all available information. An example is provided in ch 6 of volume 23, by N. Hogg. The EPR literature in this area is sparse, but will undoubtedly increase in the years ahead. In the author’s view this is a particularly promising opportunity for further improvement of the SNR in EPR spectroscopy.
4.MULTIFREQUENCY EPR
In 1963, Varian initiated a project to develop a commercial EPR bridge at Q-band (35GHz) headed by the author. The bridge circuit was the first from Varian to employ a circulator and reference arm. It was a modest commercial success – about 100 units were sold over the ensuing years. Analysis of the business opportunity presented by development of a bridge operating at a third microwave frequency, S-band, was not favorable.
After establishment of the National Biomedical EPR Center at the Medical College of Wisconsin, funds became available to develop an S-band bridge for use in collaborative projects at the center. The project was headed by Wojciech Froncisz, visiting from Jagiellonian University, Krakow, Poland. A commercially available tunable 2 to 4 GHz (octave bandwidth) microwave oscillator was used in the bridge. Although microwave cavities were initially used, the ungainly size of these structures led directly to the development of loop gap resonators.
In the ensuing years, octave bandwidth bridges were developed from 0.5 to 1, 1 to 2, and 4 to 8 GHz, resulting in continuous coverage from 0.5 to 8 GHz in coaxial transmission line configurations. An additional bridge was developed at 18 GHz, resulting in EPR capabilities at discrete frequencies in
TRENDS IN EPR TECHNOLOGY |
421 |
three narrow bands centered at 9.5, 18 and 35 GHz. This multifrequency capability was viewed as a national resource available to all EPR spectroscopists.
The 18 GHz bridge was designed to be suitable both for CW and saturation recovery (SR) EPR. An S-band module was developed for use with Froncisz’s S-band bridge, resulting in multifrequency capabilities for saturation recovery at S-, X-, and K-band (see ch. 1 in this volume, by Eaton and Eaton).
Bruker has developed an approach that may well simplify the problem of achieving pulse multifrequency capabilities. The firm’s W-band (94 GHz) bridge is based on an X-band bridge with advanced pulse capabilities. The output of the X-band bridge is mixed with the output of an 84 GHz oscillator to form a W-band microwave source, and the reflected signal from the W- band resonator is translated back to X-band for detection. The same strategy has recently been developed at Q-band, translating again from X-band, Tradeoffs made by this approach have not yet been analyzed in the literature.
Several initiatives were undertaken at MCW to arrive at multifrequency ENDOR and ELDOR capabilities in the S-band range. Newton and Hyde (1991) observed that the ENDOR enhancement effect increases inversely with the applied static magnetic field, which makes S-band a favorable microwave frequency for ENDOR of low moment nuclei such as They demonstrated
ENDOR of the P1 center in diamond. Pace et al. (1993) used S-band ENDOR to observe hyperfine interaction of
and
nuclei in trinitrophenylmethylnitroxide. Christidis et al. (1994) describe a probehead with both interchangeable LGRs and RF coils for multifrequency ENDOR in the range of 1 – 10 GHz. An ELDOR capability at S-band using two separate S-band bridges and a bimodal resonator was described by Piasecki et al. (1996).
Extensions of X-band multiquantum EPR to MQ ENDOR (Mchaourab et al., 1993) and electron-nuclear-electron triple resonance (Christidis et al., 1996) were reported. MQ ELDOR is described by Mchaourab et al. (1991). The center in Milwaukee has recently completed a Q-band bridge with conventional CW and ELDOR capability, MQ and MQ ELDOR capability and saturation recovery. Use of all five displays for ENDOR seems straightforward. The sample and resonator need not be changed when switching between modes of operation. Historically, each of these advanced capabilities was developed one at a time; it is now possible to combine them in a single microwave configuration. Translation to another microwave frequency following Bruker’s approach seems feasible, although multiquantum EPR presents a special problem. The translation processes may generate unacceptable levels of intermodulation sidebands. A possible solution to this problem would be to translate the two closely spaced
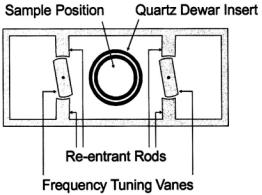
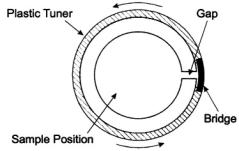
TRENDS IN EPR TECHNOLOGY |
423 |
Figure 7. Variable frequency bridged loop gap resonator.
5.EPR FOR ROUTINE ANALYSIS
The use of EPR spectroscopy in routine commercial applications began as early as 1961, when Saraceno, Fanale and Coggeshall of Gulf Oil (Saraceno et al., 1961; Saraceno, 1963) developed techniques for using EPR to measure vanadium in crude oil. The vanadium poisoned catalysts used in refineries. Under contract with Gulf, Varian Associates developed a portable EPR spectrometer with various automatic calibration features that permitted its reliable operation by unskilled personnel in the environment of a refinery (Nelson and Baker, 1967).
Another effort occurred in 1971 when President Nixon requested and Congress agreed to the establishment of a Special Action Office of Drug Abuse, primarily for compulsory treatment and rehabilitation of addicted Vietnam veterans. Syva Corporation, a cooperative venture between Syntex and Varian, developed the Frat™ EPR spin immunoassay systems to monitor morphine in the urine of addicts. The EPR instrument was highly automated and approximately 50 spectrometers were built. Many were used in extremely difficult conditions in Vietnam, making several million examinations. See Hyde (1998b) for additional details.
In 1973, Hwang and Pusey developed an oil well logging process for determining paleotemperatures (Pusey, 1973; Hwang and Pusey, 1973). They measured the EPR free radical signal that occurs naturally in kerogen. The process was made commercially available by Robertson Research International, Ltd.
One of the most promising applications for routine EPR analysis is for radiation dosimetry using the tissue-equivalent alanine dosimeter. The National Institute of Standards and Technology (NIST) offers a number of