
Biomedical EPR Part-B Methodology Instrumentation and Dynamics - Sandra R. Eaton
.pdf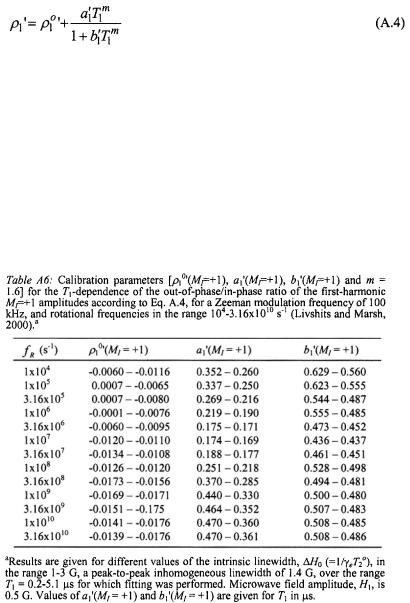
362 |
DEREK MARSH ET AL. |
The second set of calibrations is given for the out-of-phase/in-phase ratios of the first-harmonic integrated spectral intensities (Tables A3-A5) and low-field spectral amplitudes (Tables A3, A6, A7). For all calibrations, the
ratio depends on
according to the following semi-empirical expression (Livshits et al., 1998a; Livshits and Marsh, 2000):
where the parameters and
depend quite strongly on
and the Zeeman modulation frequency,
but less strongly on
and the spin label rotational rate,
The exponent m in Eq. A.4 depends on the modulation frequency and also on whether signal amplitudes or integrated intensities are measured. Table A3 gives calibrations established for the “no-motion” situation, which applies to both quasi-rigid limit and extreme motional narrowing spectra (Livshits et al., 1998a). Tables A4-A7 give corresponding calibrations that specifically take account of molecular motion (Livshits and Marsh, 2000). For the first-harmonic out-of-phase method, calibrations are given not only for the standard modulation frequency
but also for a modulation frequency of 25 kHz. Calibrations for
show enhanced sensitivity to longer
relaxation times.


364 DEREK MARSH ET AL.
Corless J.M., McCaslin D.R., Scott B.L. (1982). Two-dimensional rhodopsin crystals from disk membranes of frog retinal rod outer segments. Proc Natl Acad Sci USA 79, 11161120.
Deatherage J.F., Henderson R., Capaldi R.A. (1982) Relationship between membrane and cytoplasmic domains in cytochrome c oxidase by electron microscopy in media of different density. J Mol Biol 158, 501-514.
Downer N.W. (1985) Cross-linking of dark adapted frog photoreceptor disk membranes - evidence for monomeric rhodopsin. Biophys J 47, 285-293.
Eastman M.P., Kooser R.G., Das M.R., Freed J.H. (1969) Studies of Heisenberg spin exchange in ESR spectra. I. Linewidth and saturation effects. J Chem Phys 51, 26902709.
Esmann M., Hideg K., Marsh D. (1994) Influence of poly(ethylene glycol) and aqueous viscosity on the rotational diffusion of membranous Na, K-ATPase. Biochemistry 33, 3693-3697.
Esmann M., Marsh D. (1992) Local translational diffusion rates of membranous
measured by saturation transfer ESR spectroscopy. Proc Natl Acad Sci USA 89, 7606-7609.
Evans C.A. (1981) Use of integral of saturation transfer electron paramagnetic spectra to determine molecular rotational correlation times. Slowly tumbling spin labels in the presence of rapidly tumbling spin labels. J Magn Reson 44, 109-116.
Fajer P., Knowles P.F., Marsh D. (1989) Rotational motion of yeast cytochrome oxidase in phosphatidylcholine complexes studied by saturation-transfer electron spin resonance.
Biochemistry 28, 5634-5643.
Fajer P., Marsh D. (1983a) Analysis of dispersion mode saturation-transfer ESR spectra. Application to model membranes. J Magn Reson 55, 205-215.
Fajer P., Marsh D. (1983b) Sensitivity of saturation transfer ESR spectra to anisotropic rotation. Application to membrane systems. J Magn Reson 51, 446-459.
Fajer P., Thomas D.D., Feix J.B., Hyde J.S. (1986) Measurement of rotational molecular motion by time-resolved saturation transfer electron paramagnetic resonance. Biophys J 50, 1195-1202.
Halbach K. (1954) Über eine neue Methode zur Messung von Relaxationszeiten und über den Spin von Helv Phys Acta 27, 259-282.
Hidalgo C., Thomas D.D., Ikemoto N. (1978) Effect of the lipid environment on protein motion and enzymatic activity of the sarcoplasmic reticulum calcium ATPase. J Biol Chem 253, 6879-6887.
Hoffmann W., Pink D.A., Restall C., Chapman D. (1981) Intrinsic molecules in fluid phospholipid bilayers. Fluorescence probe studies. Eur J Biochem 114, 585-589.
Holzenburg A., Jones P.C., Franklin T., Páli T., Heimburg T., Marsh D., Findlay J.B.C., Finbow M.E. (1993) Evidence for a common structure for a class of membrane channels.
Eur J Biochem 213, 21-30.
Horváth L.I., Brophy P.J., Marsh D. (1988) Influence of lipid headgroup on the specificity and exchange dynamics in lipid-protein interactions. A spin label study of myelin proteolipid apoprotein-phospholipid complexes. Biochemistry 27, 5296-5304.
Horváth L.I., Brophy P.J., Marsh D. (1993) Exchange rates at the lipid-protein interface of the myelin proteolipid protein determined by saturation transfer electron spin resonance and
continuous wave saturation studies. Biophys J 64, 622-631. |
|
|
Horváth L.I., Dux L., Hankovszky H.O., Hideg K., Marsh D. (1990) |
Saturation transfer |
|
electron spin resonance of |
covalently spin-labeled with |
vinyl |
ketoneand maleimide-nitroxide derivatives. Effects of segmental motion and labeling levels. Biophys J 58, 231-241.

SATURATION TRANSFER SPECTROSCOPY |
365 |
Horváth L.I., Marsh D. (1988) Improved numerical evaluation of saturation transfer electron spin resonance spectra. J Magn Reson 80, 314-317.
Horváth L.I., Marsh D. (1983) Analysis of multicomponent saturation transfer ESR spectra using the integral method: application to membrane systems. J Magn Reson 54, 363-373.
Hubbell W.L., Altenbach C. (1994) “Site-directed spin-labeling of membrane proteins.” In
Membrane protein structure: experimental approaches., White S.H., ed. New York: Oxford University Press, pp. 224-248.
Hyde J.S. (1978) “Saturation-transfer spectroscopy.” In Methods in Enzymology, Hirs C.H.W., Timasheff S.N., eds. New York: Academic Press, pp. 480-511.
Hyde J.S., Dalton L. (1972) Very slowly tumbling spin labels: adiabatic rapid passage. Chem Phys Lett 16:568-572.
Hyde J.S., Dalton L.R. (1979) “Saturation-transfer spectroscopy.” In Spin-Labeling II. Theory and Applications, Berliner L.J., ed. New York: Academic Press, pp. 71-113.
Hyde J.S., Eriksson L.E.G., Ehrenberg A. (1970) EPR relaxation of slowly moving flavin radicals: “Anomalous” saturation. Biochim Biophys Acta 222, 688-692.
Hyde J.S., Subczynski W.K. (1989) “Spin-label oximetry.” In Spin Labeling. Theory and Applications, Berliner L.J., Reuben J., eds. New York and London: Plenum Press, pp. 399425.
Hyde J.S., Thomas D.D. (1973) New EPR methods for the study of very slow motion:
application to spin-labeled hemoglobin. Ann N Y Acad Sci 222, 680-692. |
|
Jesaitis A.J., Yguerabide J. (1986) The lateral mobility of the |
ATPase in |
Madin-Darby canine kidney cells. J Cell Biol 102, 1256-1263. |
|
Johnson M.E., Hyde J.S. (1981) 35-GHz (Q-band) saturation transfer electron paramagnetic resonance studies of rotational diffusion. Biochemistry 20, 2875-2880.
Kirino Y., Ohkuma T., Shimizu H. (1978) Saturation transfer electron spin resonance study on the rotational diffusion of calciumand magnesium-dependent adenosine triphosphatase in sarcoplasmic reticulum membranes. J Biochem (Tokyo) 84, 111-115.
Knowles P.F., Watts A., Marsh D. (1979) Spin label studies of lipid immobilization in dimyristoylphosphatidylcholine-substituted cytochrome oxidase. Biochemistry, 18, 44804487.
Kurad D., Jeschke G., Marsh D. (2001) Spin-label HF-EPR of lipid ordering in cholesterolcontaining membranes. Appl Magn Reson 21, 469-481.
Kusumi A., Hyde J.S. (1982) Spin-label saturation-transfer electron spin resonance detection of transient association of rhodopsin in reconstituted membranes. Biochemistry 21, 59785983.
Kusumi A., Ohnishi S., Ito T., Yoshizawa T. (1978) Rotational motion of rhodopsin in the visual receptor membrane as studied by saturation-transfer spectroscopy. Biochim Biophys Acta 507, 539-543.
Langen R., Isas J.M., Luecke H., Haigler H.T., Hubbell W.L. (1998) Membrane-mediated assembly of annexins studied by site-directed spin labeling. J Biol Chem 273, 2245322457.
Leigh Jr. J.S. (1970) ESR rigid-lattice line shape in a system of two interacting spins. J Chem Phys 52, 2608-2612.
Livshits V.A. (1974) Slow anisotropic tumbling in ESR spectra of nitroxyl radicals. J Magn Reson 24, 307-313.
Livshits V.A., Dzikovski B.G., Marsh D.(2001) Mechanism of relaxation enhancement of spin labels in membranes by paramagnetic ion salts: dependence on 3d and 4f ions and on the anions. J Magn Reson 148, 221-237.
Livshits V.A., Dzikovski B.G., Marsh D. (2003) Anisotropic motion effects in CW non-linear EPR spectra. Spin relaxation enhancement of lipid spin labels. J Magn Reson, in press.
366 DEREK MARSH ET AL.
Livshits V.A., Marsh D. (2000) Spin relaxation measurements using first-harmonic out-of- phase absorption EPR signals: rotational motion effects. J Magn Reson 145, 84-94.
Livshits V.A., Páli T., Marsh D. (1998a) Spin relaxation measurements using first-harmonic out-of-phase absorption EPR signals. J Magn Reson 134, 113-123.
Livshits V.A., Páli T., Marsh D. (1998b) Relaxation time determinations by progressive saturation EPR: Effects of molecular motion and Zeeman modulation for spin labels. J Magn Reson 133, 79-91.
Marsh D. (1980) Molecular motion in phospholipid bilayers in the gel phase: long axis rotation. Biochemistry 19, 1632-1637.
Marsh D. (1990) Sensitivity analysis of magnetic resonance spectra from unoriented samples.
J Magn Reson 87, 357-362.
Marsh D. (1992a) Influence of nuclear relaxation on the measurement of exchange frequencies in CW saturation EPR studies. J Magn Reson 99, 332-337.
Marsh D. (1992b) Exchange and dipolar spin-spin interactions and rotational diffusion in saturation transfer EPR spectroscopy. Appl Magn Reson 3, 53-65.
Marsh D. (1995) Sensitivity of the continuous wave saturation of conventional EPR spectra to slow rotational motion. Spectrochim Acta 51A, 111-114.
Marsh D., Horváth L.I. (1989) “Spin-label studies of the structure and dynamics of lipids and proteins in membranes.” In Advanced EPR. Applications in Biology and Biochemistry,
Hoff A.J., ed. Amsterdam: Elsevier, pp. 707-752.
Marsh D., Horváth L.I. (1992a) A simple analytical treatment of the sensitivity of saturation transfer EPR spectra to slow rotational diffusion. J Magn Reson 99, 323-331.
Marsh D., Horváth L.I. (1992b) Influence of Heisenberg spin exchange on conventional and phase-quadrature EPR lineshapes and intensities under saturation. J Magn Reson 97, 1326.
Marsh D., Horváth L.I. (1998) Structure, dynamics and composition of the lipid-protein interface. Perspectives from spin-labelling. Biochim Biophys Acta 1376, 267-296.
Marsh D., Kurad D., Livshits V.A. (2002) High-field electron spin resonance of spin labels in membranes. Chem Phys Lipids 116, 93-114.
Marsh D., Livshits V.A., Páli T. (1997) Non-linear, continuous-wave EPR spectroscopy and spin-lattice relaxation: spin-label EPR methods for structure and dynamics. J Chem Soc Perkin Trans 2, 2545-2548.
Marsh D., Páli T., Horváth L.I. (1998) “Progressive saturation and saturation transfer EPR for measuring exchange processes and proximity relations in membranes.” In Spin Labeling. The Next Millenium., Berliner L.J., ed. New York: Plenum Press, pp. 23-82.
Maunsbach A.B., Skriver E., Soderholm M., Hebert H. (1989) “Three-dimensional structure and topography of membrane bound Na,K-ATPase.” In The Na,K-Pump. Part A: Molecular Aspects. Progress in Clinical and Biological Research., Skou J.C., Norby J.G., Maunsbach A.B., Esmann M., eds. New York: Alan Liss, pp. 39-56.
McLaughlin A., Gratwohl G., McLaughlin S. (1978) The adsorption of divalent cations to phosphatidylcholine bilayer membranes. Biochim Biophys Acta 513, 338-357.
Molin Y.N., Salikov K.M., Zamaraev K.I. (1980) Spin Exchange. Principles and Applications in Chemistry and Biology. Berlin: Springer Verlag.
Páli T., Bartucci R., Horváth L.I., Marsh D. (1992) Distance measurements using paramagnetic ion-induced relaxation in the saturation transfer electron spin resonance of spinlabeled biomolecules. Application to phospholipid bilayers and interdigitated gel phases. Biophys J 61, 1595-1602.
Páli T., Finbow M.E., Marsh D. (1999) Membrane assembly of the 16-kDa proteolipid channel from Nephrops norvegicus studied by relaxation enhancements in spin-label ESR. Biochemistry 38, 14311 -14319.
SATURATION TRANSFER SPECTROSCOPY |
367 |
Páli T., Horváth L.I., Marsh D. (1993) Continuous-wave saturation of two-component, inhomogeneously broadened, anisotropic EPR spectra. J Magn Reson A101, 215-219.
Páli T., Livshits V.A., Marsh D. (1996) Dependence of saturation-transfer EPR intensities on spin-lattice relaxation. J Magn Reson B 113, 151-159.
Portis A.M. (1955) Rapid passage in electron spin resonance. Phys Rev 100, 1219-1224. Robinson B.H., Dalton L.R. (1980) Anisotropic rotational diffusion studied by passage
saturation transfer electron paramagnetic resonance. J Chem Phys 72, 1312-1324. Rousselet A., Devaux P.F. (1977) Saturation transfer electron paramagnetic resonance on
membrane bound proteins. II. Absence of rotational diffusion of the cholinergic receptor protein in Torpedo marmorata membrane fragments. Biochem Biophys Res Commun 78, 448-454.
Ryba N.J.P., Marsh D. (1992) Protein rotational diffusion and lipid/protein interactions in recombinants of bovine rhodopsin with saturated diacylphosphatidylcholines of different chain lengths studied by conventional and saturation transfer electron spin resonance.
Biochemistry 31, 7511-7518.
Saffman P.G., Delbrück M. (1976) Brownian motion in biological membranes. Proc Natl Acad Sci USA 72, 3111-3113.
Slichter, C. P. (1978) Principles of Magnetic Resonance. Berlin-Heidelberg-New York: Springer-Verlag,.
Snel M.M.E., Marsh D. (1994) Membrane location of apocytochrome c and cytochrome c determined from lipid-protein spin exchange interactions by continuous wave saturation electron spin resonance. Biophys J 67, 737-745.
Solomon I. (1955) Relaxation processes in a system of 2 spins. Phys Rev 99, 559-565.
Squier T.C., Thomas D.D. (1986) Methodology for increased sensitivity and precision in saturation transfer electron paramagnetic resonance studies of molecular dynamics. Biophys J 49, 921-935.
Tardieu A. (1972) Thesis/Dissertation. Université de Paris-Sud..
Thomas D.D., Dalton L.R., Hyde J.S. (1976) Rotational diffusion studied by passage saturation transfer electron paramagnetic resonance. J Chem Phys 65, 3006-3024.
Thomas D.D., McConnell H.M. (1974) Calculation of paramagnetic resonance spectra sensitive to very slow rotational motion. Chem Phys Lett 25, 470-475.
Thomas D.D., Seidel J.C., Hyde J.S., Gergely J. (1975) Motion of subfragment-1 in myosin and its supramolecular complexes: saturation transfer electron paramagnetic resonance.
Proc Natl Acad Sci USA 72, 1729-1733.
Weger M. (1960) Passage effects in paramagnetic resonance experiments. Bell Syst Tech J 39, 1013-1112.
Yin J.J., Hyde J.S. (1987) Application of rate equations to ELDOR and saturation recovery experiments on N-14-N-15 spin label pairs. J Magn Reson 74, 82-93.
370 ALBERT H. BETH AND ERIC J. HUSTEDT
membranes. EPR, in particular, has provided unique characterization of the structure and dynamics of the lipids that solvate the periphery of intrinsic membrane proteins, the so-called boundary lipids (e.g. Griffith and Jost, 1976; Ge and Freed, 1993; Borbat et al., 2001). Most recently, these basic techniques and in particular EPR have been utilized to investigate the lateral organization of lipids in cell membranes including the sizes and stabilities of microdomains (e.g. Kawasaki et al., 2001). These investigations have taken on added importance with the discovery of specialized structures including lipid “rafts” that are hypothesized to play important roles in modulating protein-membrane interactions and in cellular signaling pathways (e.g. Zacharias et al., 2002; Anderson and Jacobson, 2002).
Less is known about the organization and dynamics of intrinsic membrane proteins despite the central role that they play in regulating cellular functions. Studies of membrane protein global dynamics are particularly challenging due in large part to the anisotropic, viscous nature of the bilayer, the number of different proteins that are present, and the very high local concentrations of total protein. The latter property merits serious attention. While there is variability in the percentage by weight of lipid and protein in different specialized membranes (e.g. myelin versus inner mitochondrial), the plasma membranes of cells and many subcellular organelle membranes are often composed of roughly equal percentages of lipid and protein by weight. Using average values for molecular weights and sizes of lipids and intrinsic membrane proteins, on the order of 30% of the outer membrane surface area can be occupied by protein and the effective concentration of protein within the volume of the bilayer can exceed 100 mg/mL! Given this extremely crowded two-dimensional environment, there are obvious questions regarding the extent of weak interactions between membrane proteins that would not be observed following extraction with non-denaturing detergents and how such interactions might modulate the functions of membrane proteins. Currently, there is unprecedented interest in determining the static structures of membrane proteins and in understanding their functional dynamics. As the structural database of individual membrane proteins continues to expand, studies designed to determine the structural consequences of interactions between proteins in the crowded environment of the cell membrane will undoubtedly take on increased importance.
A number of different experimental approaches have been utilized to examine the oligomeric state and higher order lateral organization of membrane proteins in situ including chemical cross-linking, visualization by freeze-fracture electron microscopy, visualization by fluorescence microscopy, and target size analysis by neutron inactivation to cite a few. Each of these approaches has provided valuable information but each is limited in its ability to provide information on dynamic aspects of protein-
SATURATION TRANSFER EPR |
371 |
protein interactions under native conditions. Fluorescence resonance energy transfer (FRET; Stryer, 1978) between labeled subunits has been utilized extensively to provide direct information on the stable assembly and dynamic proximity of membrane proteins (e.g. Blackman et al., 1998) including changes in assembly associated with cellular signaling events in living cells (e.g. Martin-Fernandez et al., 2002).
Studies of the translational or rotational dynamics of membrane proteins have been carried out under a wide range of experimental conditions including in living cells and these studies have provided important insights into static and dynamic aspects of their assembly and interactions. Most studies of translational diffusion have utilized fluorescence recovery after photobleaching (FRAP; Frye and Edidin, 1970; Webb, 1981) due in large part to the excellent signal-to-noise ratio and dynamic range that this methodology provides. More recently, single particle tracking experiments have provided new insights into the constraints on the lateral motion of membrane proteins (e.g. Cherry et al., 1998). While these optical techniques provide outstanding capabilities for investigating the molecular basis for constraints to the long range lateral movement of membrane proteins on the cell surface (e.g. Koppel et al., 1981), the translational diffusion coefficient is weakly dependent on the effective size of the diffusing species (Saffman and Delbrück, 1975) and hence, to oligomeric state or to other localized, transient interactions. On the other hand, the rotational diffusion coefficient,
of an integral membrane protein undergoing global uniaxial rotational diffusion (URD) is inversely proportional to the square of the cross sectional radius of the protein complex (Saffman and Delbrück, 1975; Jähnig, 1986). Thus, measurements of rotational diffusion should be very sensitive to the presence of homo and hetero oligomeric protein complexes in the membrane.
Given the predicted high sensitivity of the correlation time for URD, which can be defined as on the effective sizes of membrane proteins and their complexes in membranes, it is not surprising that considerable effort during the past three decades has been devoted to developing spectroscopic methods that can measure their rotational dynamics in systems ranging from reconstituted proteoliposomes to intact, living cells (Edidin, 1974). These efforts have led to the development of two complementary approaches that have been successfully applied to measuring the very slow rotational dynamics, on the timescale of
to msec, that are characteristic of many integral membrane proteins in their natural membranes or in proteoliposomes.
The first approach is a collection of related optical methods that measure the transient optical anisotropy (TOA) or dichroism, following a short excitation pulse of light, of long lived triplet probes coupled to a target
372 ALBERT H. BETH AND ERIC J. HUSTEDT
protein (Cone, 1972; Naqvi et al., 1973; Cherry et al., 1976; Cherry and Schneider, 1976). TOA methods and their applications to measure the rotational diffusion of membrane proteins have been reviewed previously (e.g. Cherry, 1978; 1979; 1981; Thomas et al., 1985). Analytical expressions have been derived for the amplitudes and rates of anisotropy decays for a variety of diffusion models including URD (Cherry, 1979), constrained URD (Wahl, 1975, Szabo, 1984), wobble in a cone (Szabo, 1984), and generalized anisotropic diffusion (Weber, 1971; Chuang and Eisenthal, 1972; Belford et al., 1972; Ehrenberg and Rigler, 1972). These various models provide a sound theoretical base upon which experimental data can be analyzed in a model dependent fashion to determine the values of of membrane proteins as well as the number of different rotational species that are present. Unique interpretation of TOA data from anisotropic systems requires determination of the orientation of the molecular probe reference frame relative to the molecular reference frame and the uniqueness of this orientation. Determination of the orientations of the absorption and emission dipoles of a chromophore relative to the molecular reference frame can be quite challenging (e.g. Blackman et al., 1996) in comparison with the relative ease with which the orientation of a spin label can be determined by EPR (e.g. Hustedt and Beth, 1996).
Typically, TOA data are analyzed by fitting to a multi-exponential decay curve. The biggest challenge in interpreting the anisotropy decays from membrane proteins is the assignment of the multiple decay components to distinct molecular species and to specific dynamic processes. Even for simple unconstrained URD, two decay components are predicted for each distinct oligomeric species that is present (Nigg and Cherry, 1980). Experimentally, one often observes many decay components and it is possible to fit the complex decays to a variety of different multi-component models with similar statistical agreement between experiment and theory. Matayoshi and Jovin (1991) and Blackman et al., (1996; 2001) have addressed some of the complexities that are involved in uniquely interpreting TOA data from membrane proteins in cell membranes.
The second approach that has been utilized to characterize the rotational diffusion of intrinsic membrane proteins is an EPR method, developed by Hyde and Dalton (1972) which measures the effects of rotational diffusion on spectral lineshapes from nitroxide spin labeled proteins under conditions of continuous, partially saturating microwave excitation. The EPR method, which derives its sensitivity to motions from the “transfer” of microwave “saturation” between different orientational resonance positions of the spectrum due to rotational diffusion, has been universally referred to as saturation transfer EPR (ST-EPR; Thomas et al., 1976). A number of reviews of ST-EPR and its applications to studies of very slow rotational