
Biomedical EPR Part-B Methodology Instrumentation and Dynamics - Sandra R. Eaton
.pdfSATURATION TRANSFER EPR |
393 |
Loop-gap resonators also have very favorable field characteristics for STEPR (reviewed in Hyde and Froncisz, 1989). It remains an important goal to design modulation coils that will improve
field and phase homogeneity over the dimensions of most samples that are studied using ST-EPR. Until further advances are made with regards to homogeneity of each of these parameters, there will be some systematic error in determining correlation times from nonlinear least squares fitting of experimental data and some loss of discrimination in comparing the accuracy of different rotational diffusion models.
Previous work has shown that global analysis of ST-EPR data sets obtained at different microwave and modulation frequencies enables determination of rotational diffusion coefficients for membrane proteins, testing of the adequacy of different rotational diffusion models, determination of the number of different overlapping motional species that are present, and assessment of the confidence levels for each recovered parameter (e.g. Hustedt and Beth, 1995; 2001; Blackman et al., 2001; Stein et al., 2002). One of the strengths of global analysis is the ability to simultaneously optimize the fit of a large number of different experiments (see Beechem, 1992; Hustedt et al., 1993). Previous work has shown that several EPR signals, both inand out-of-phase and at the first and second harmonics of the modulation as well as dispersion signals, are sensitive to the correlation time for rotational diffusion (e.g Perkins et al., 1976). The
signal has been utilized extensively in past ST-EPR studies due to its sensitivity to rotational dynamics in the
to msec correlation time range, its favorable signal-to-noise ratio relative to the
signal when using a conventional cavity, and the capability of most commercial spectrometers to record this signal. Recent advances including time-locked subsampling (TLSS; Hyde et al., 1998) have enabled the simultaneous recording of all eight unique signals at the first and second harmonics of the modulation with preservation of the relative amplitudes of the individual signals. Given that the computational approaches that have been developed also calculate these same signals (Robinson and Dalton, 1980; Hustedt and Beth, 1995; 2001), it seems logical to explore what additional information can be gained from global analysis of all eight signals and their relative amplitudes rather than discarding all of the signals except
Recent advances in resonator design and in the development of low phase-noise microwave oscillators permit the routine detection of dispersion signals with excellent signal-to-noise ratios as described in detail in the chapter by Hyde in this volume (ch. 13).
Digital detection also provides a convenient way to overcome problems of proper selection of the modulation phase for detecting true out-of-phase signals etc.) Automated methods have been developed that permit post-processing of the data for accurate phasing of the signals once they
394 |
ALBERT H. BETH AND ERIC J. HUSTEDT |
have been recorded digitally (Auteri et al., 1988). This advance circumvents the need to carry out time-consuming self-nulling of the signals (see Thomas et al., 1976) prior to phase sensitive detection.
There are a number of intrinsic membrane proteins that contain only a single membrane spanning These include proteins like the glycophorins in erythrocytes and the growth factor receptors including the ErB family. The rotational motions of these proteins have been difficult to measure due to the fact that they exhibit correlation times in the submicrosecond time window. Rotational motions in the 100 nsec to
time window are difficult to measure using conventional time-resolved optical approaches due to the nsec fluorescent lifetimes of most fluorophores and the difficulties of PMT gating and rejection of photons from the tail of the laser pulse in phosphorescence experiments (e.g. Herman et al., 1992). Linear EPR provides very limited sensitivity to motion in this time window (McCalley et al., 1972). Using standard 50 kHz field modulation, the
STEPR signal also shows very limited sensitivity to the correlation time for isotropic motion in this range (Thomas et al., 1976) and URD with modulation frequencies up to 100 kHz shows low sensitivity (Stein et al., 2002). While the
ST-EPR signal shows good sensitivity to motion in this range (Hyde and Dalton, 1972), it has a greatly reduced amplitude relative to that observed at correlation times longer than
This reduction in amplitude, coupled with the difficulty in obtaining
ST-EPR signals using conventional cavities at X-band, has precluded its use in most studies of membrane protein dynamics. Even though ST-EPR can provide a signal-to- noise ratio that is comparable to linear EPR, most applications of the technique in cells and in intact membrane preparations require long data acquisition times to obtain high quality data due to the low concentration of protein in a loosely packed suspension of cells or membranes (even though the concentration within the bilayer is high, the concentration per unit volume of cells or intact membranes is low due to the large intracellular and extracellular aqueous compartments that contain no protein).
The calculated spectra in Figure 10 suggest that it might be possible to increase the sensitivity of the signal to motion in this time window by utilizing higher modulation frequencies. Currently available commercial spectrometers do not provide the capability of recording
signals at frequencies higher than 100 kHz. Undoubtedly, this has limited experimental testing of the potential advantages of higher modulation frequencies in previous applications of the technique.
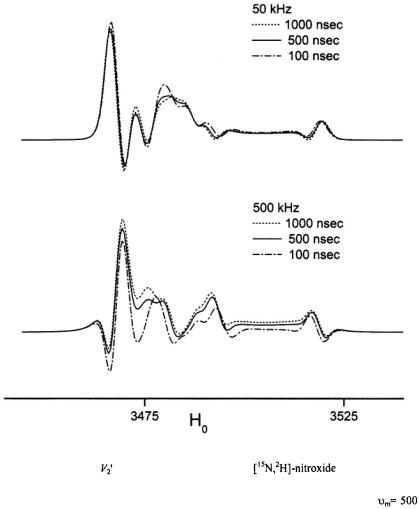
SATURATION TRANSFER EPR |
395 |
Figure 10. Calculated X-band ST-EPR spectra for a |
undergoing URD |
with and
(dotted line),
(solid line), and
(dashed dotted line) for modulation frequencies of (upper panel) and
(lower panel).
396 |
ALBERT H. BETH AND ERIC J. HUSTEDT |
6.APPLICATIONS OF ST-EPR TO MEMBRANE PROTEINS
6.1Applications Based upon Isotropic Model System Analyses
ST-EPR has been utilized to characterize the rotational dynamics of a wide range of intrinsic and peripheral membrane proteins including: CaATPase in sarcoplasmic reticulum, rhodopsin, mitochondrial electron transport enzymes, cytochrome P-450, acetyl choline receptor, and a number of erythrocyte membrane proteins including band 3 (AE1), ankyrin, spectrin, hemoglobin, and GAPDH (see Thomas, 1985, for a comprehensive review of these early studies). Since that time, studies have been reported on a variety of membrane proteins including: erythrocyte anion exchange protein (AE1; Beth et al, 1986; Anjaneyulu et al., 1988; 1989; Cobb et al., 1990); cytochrome c reductase (Gwak et al., 1986); Ca-ATPase (e.g. Bigelow and Thomas, 1987; Squier et al., 1988; Squier et al., 1988; Lewis and Thomas, 1991; Mahaney and Thomas, 1991; Cornea and Thomas, 1994; Negash et al., 1996); Na,K-ATPase (Mahaney et al., 1990; Mahaney and Grisham, 1992; Esmann et al., 1992); cytochrome P-450’s (Schwarz et al., 1990; 1999); cytochrome c oxidase (Qiu et al., 1992); EGF receptor (Rousseau et al., 1993); H/K-ATPase (Middleton et al., 1995); spectrin (Fung et al., 1996); bacteriophage M13 coat protein (Wolkers et al., 1997); and GalP (Marsh and Henderson, 2001). Effective rotational correlation times for the proteins examined in these studies have been determined by comparison of motion sensitive ratio parameters with those obtained from isotropic model systems such as spin labeled hemoglobin (Thomas et al., 1976) or spin labeled GAPDH (Beth et al., 1981) or by fitting experimental ST-EPR spectra based on an isotropic rotational diffusion model (Rousseau et al., 1993). Collectively, these studies have demonstrated the very slow rotational diffusion of membrane proteins that has been predicted by hydrodynamic theory (Saffman and Delbruck, 1975; Jahnig, 1986) and they have provided important qualitative insights into changes in rotational dynamics that are related to biological function.
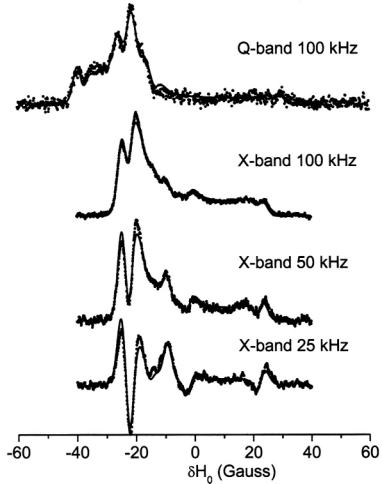
398 |
ALBERT H. BETH AND ERIC J. HUSTEDT |
Three recent ST-EPR studies of membrane protein rotational dynamics have taken advantage of the currently available computational algorithms to analyze experimental data. In the first study, Hustedt and Beth (1995) utilized the global analysis approach (Hustedt et al., 1993) to determine the simultaneous best-fit of the ST-EPR signals obtained at modulation frequencies of 25, 50, and 100 kHz from spin labeled AE1 (band 3) in human erythrocytes. The analyses indicated that the data at all three modulation frequencies could be simultaneously fit with a URD model with a single rotational correlation time of
and a tilt of the spin label relative to the URD axis of 39°. Attempts to improve the statistics
of the fits by utilizing more than one URD population were unsuccessful. At the time, these results were controversial since previous TOA studies had suggested that multiple rotational sub-populations of AE1 existed in the erythrocyte membrane and that as much as 75% of AE1 was highly clustered and characterized by rotational correlation times in the msec time range or longer (e.g. Corbett et al., 1994; see also Matayoshi and Jovin, 1991; Blackman et al., 1998; Blackman et al., 2001). In subsequent work, Hustedt and Beth (1996) determined the orientation of the spin label employed in these studies relative to the membrane normal axis by orienting erythrocytes by flow in an EPR flat cell. These studies showed that the z-axis of the nitroxide was tilted from the membrane normal axis by 38°. Since URD of intrinsic membrane proteins is predicted to occur about an axis that is parallel with the membrane normal, this result reinforced the conclusions obtained from analysis of the ST-EPR data (Hustedt and Beth, 1995). More recent work has shown that global analysis of X-band data obtained at modulation frequencies of 25, 50, and 100 kHz along with Q-band data obtained at a modulation frequency of 100 kHz is best fit by a single unconstrained URD model with a correlation time of
and a major tilt angle of 39° (Figure 11) thereby adding additional support to the previous data analysis carried out at X-band alone.
In the second study, the data from spin labeled AE1 was reevaluated using a constrained URD model (Blackman et al., 2001). Previous TOA studies had suggested that a subpopulation of AE1 might exhibit restricted amplitude rotational diffusion due to interaction of its cytoplasmic domain with the membrane skeleton (e.g. Nigg and Cherry, 1980). In these studies, a major change in anisotropy decay (e.g. a significant reduction in was observed following proteolytic cleavage of the link between the transmembane and cytoplasmic domains of AE1, a result that was consistent with a release of a constraint on the amplitude of URD of that subpopulation of AE1 that was interacting with the membrane skeleton (see also Matayoshi and Jovin, 1991; Blackman et al., 1996; 2001). Blackman et al (2001) determined that: 1) 28 % of the copies of AE1 interact with the membrane
SATURATION TRANSFER EPR |
399 |
skeleton in intact erythrocyte membranes; 2) there was no detectable change in the ST-EPR signals recorded at X- and Q-band microwave frequencies following cleavage of the cytoplasmic domain with trypsin; and 3) these STEPR results are entirely consistent with a weak constraint of 71° that was determined from the analysis of the TOA data.
In the third study, Stein et al (2002) employed X-band ST-EPR to characterize the rotational diffusion of spin labeled EGF (epidermal growth factor) bound to its receptor in A-431 membrane vesicles. Spectra recorded at modulation frequencies of 60, 80, and 100 kHz were globally analyzed using a one or a two component unconstrained URD model. These analyses indicated that the experimental data was best fit with a two-component model characterized by correlation times of 0.12 and and with different orientations of the spin label relative to the URD axis. The correlation times recovered from the analyses were reasonably consistent with those predicted for monomeric and dimeric EGF receptors and they provided a possible explanation for the known existence of high and low affinity receptors in the membrane.
These three studies demonstrate that the computational algorithms described in this chapter can be employed to analyze experimental ST-EPR data obtained from membrane proteins. These analyses have shown that it is possible to extract rotational correlation times from the data and that the adequacy of rotational diffusion models can be evaluated. With these computational tools, ST-EPR provides an important complement to TOA for detailed studies of the rotational diffusion of membrane proteins. These same computational tools should also be useful for analyzing the experimental data from other systems that exhibit URD.
6.3Future Prospects
The past decade has seen an amazing emphasis on determining the static structures of intrinsic membrane proteins using a wide range of experimental techniques. This emphasis will undoubtedly continue in the next decade given the central role that membrane proteins play in regulating cellular functions under normal physiological conditions and the number of membrane proteins that are candidates as specific drug targets in a wide range of pathological settings. As the data base of atomic resolution static structures of membrane proteins expands, studies of their functional dynamics and their interactions within cell membranes will take on added importance. The advances in site-directed spin labeling of membrane proteins that have taken place in the past decade (for recent reviews see: Hubbell et al., 1996; Feix and Klug, 1998; Hustedt and Beth, 1999; Hubbell et al., 2000; Columbus and Hubbell, 2002) places ST-EPR in a unique
SATURATION TRANSFER EPR |
401 |
7.REFERENCES
Anderson, J.R., Mett, R.R., and Hyde, J.S. (2002). Cavities with axially uniform fields for use in electron paramagnetic resonance. II. Free space generalization. Rev. Sci. Instrum., 73, 3027-3037.
Anderson, R.G.W., and Jacobson, K. (2002). A role for lipid shells in targeting proteins to caveoli, rafts, and other lipid domains. Science, 296, 1821-1825.
Anjaneyulu, P.S.R., Beth, A.H., Sweetman, B.J., Faulkner, L.A., and Staros, J.V. (1988).
a stable isotopesubstituted, membrane-impermeant bifunctional spin label for studies of the dynamics of membrane proteins: Application to the anion exchange channel in intact human erythrocytes. Biochemistry 27, 6844-6851.
Anjaneyulu, P.S.R., Beth, A.H., Cobb, C.E., Juliao, S.F., Sweetman, B.J., and Staros, J.V. (1989). Bis(sulfo-N-succinimidyl) doxyl-2-spiro-5’-azelate: Synthesis, characterization, and reaction with the anion-exchange channel in intact human erythrocytes. Biochemistry 28, 6583-6590.
Auteri, F.P., Beth, A.H., and Robinson, B.H. (1988). Finding the proper phase in CW-EPR spectroscopy: An automated method. J. Magn. Reson. 80, 493-501.
Beechem, J.M. (1992). Global analysis of biochemical and biophysical data. Methods Enzymol 210, 37-54.
Belford, G.G., Belford, R.L., and Weber, G. (1972). Dynamics of fluorescence polarization in macromolecules. Proc. Natl. Acad. Sci. USA, 69, 1392-1393.
Beth, A.H., Venkataramu, S.D., Balasubramanian, K., Dalton, L.R., Robinson, B.H., Pearson, D.E., Park, C.R. and Park, J.H. (1981). maleimide spin labels: Improved sensitivity and resolution for biological EPR studies. Proc. Natl. Acad. Sci. 78, 967-971.
Beth, A.H., Balasubramanian, K., Robinson, B.H., Dalton, L.R., Venkataramu, S.D., and Park, J.H. (1983). Sensitivity of saturation transfer electron paramagnetic resonance signals to anisotropic rotational diffusion with [15N]nitroxide spin-labels. Effects of noncoincident magnetic and diffusion tensor principal axes. J. Phys. Chem. 87, 359-367.
Beth, A.H., Conturo, T.E., Venkataramu, S.D., and Staros, J.V. (1986). Dynamics and interactions of the anion channel in intact human erythrocytes: An electron paramagnetic resonance spectroscopic study employing a new membrane-impermeant bifunctional spin label. Biochemistry 25, 3824-3832.
Beth, A.H., and Robinson, B.H. (1989). Nitrogen-15 and deuterium substituted spin labels for studies of very slow rotational motion. In: Biological Magnetic Resonance, Vol. 8, Spin Labeling - Theory and Applications, L.J. Berliner and J. Reuben, eds., Plenum Press, New York, pp. 179-253.
Bigelow, D.J., and Thomas, D.D. (1987). Rotational dynamics of lipid and the Ca-ATPase in sarcoplasmic reticulum. The molecular basis of activation by diethyl ether. J. Biol. Chem. 262, 13449-13456.
Blackman, S.M., Cobb, C.E., Beth, A.H., and Piston, D.W. (1996). The orientation of eosin- 5-maleimide on human erythrocyte band 3 measured by fluorescence polarization microscopy. Biophys. J. 71, 194-208.
Blackman, S.M., Piston, D.W., and Beth, A.H. (1998). Oligomeric state of human erythrocyte band 3 measured by fluorescence resonance energy homotransfer. Biophys. J. 75, 11171130.
Blackman, S.M., Hustedt, E.J., Cobb, C.E., and Beth, A.H. (2001). Flexibility of the cytoplasmic domain of the anion exchange protein, band 3, in human erythrocytes. Biophys. J. 81, 3363-3376.

402 ALBERT H. BETH AND ERIC J. HUSTEDT
Borbat, P.P., Costa-Filho, A.J., Earle, K.A., Moscicki, J.K., and Freed, J.H. (2001). Electron spin resonance in studies of membranes and proteins. Science 291, 266-269.
Cherry, R.J., Cogoli, A., Oppliger, M., Schneider, G., and Semenza, G. (1976). A spectroscopic technique for measuring slow rotational diffusion of macromolecules. 1. Preparation and properties of a triplet probe. Biochemistry 15, 3653-3656.
Cherry, R.J., and Schneider, G. (1976). A spectroscopic technique for measuring slow rotational diffusion of macromolecules. 2. Determination of rotational correlation times of proteins in solution. Biochemistry 15, 3657-3661.
Cherry, R.J. (1978). Measurement of protein rotational diffusion in membranes by flash photolysis. Methods Enzymol. 54, 47-61.
Cherry, R.J. (1979). Rotational and lateral diffusion of membrane proteins. Biochim. Biophys. Acta 559, 289-327.
Cherry, R.J., Smith, P.R., Morrison, I.E., and Fernandez, N. (1998). Mobility of cell surface receptors: a re-evaluation. FEBS Lett. 430, 88-91.
Cherry, R.J., and Godfrey, R.E. (1981). Anisotropic rotation of bacteriorhodopsin in lipid membranes. Comparison of theory with experiment. Biophys. J. 36, 257-276.
Chuang, T.L., and Eisenthal, K.B. (1972). Theory of fluorescence depolarization by anisotropic rotational diffusion. J. Chem. Phys. 57, 5094-5097.
Cobb, C.E., Juliao, S., Balasubramanian, K., Staros, J.V., and Beth, A.H. (1990). Effects of diethyl ether on membrane lipid ordering and on rotational dynamics of the anion exchange protein in intact human erythrocytes: Correlations with anion exchange function. Biochemistry 29, 10799-10806.
Coffey, P., Robinson, B.H., and Dalton, L.R. (1976). Rapid computer simulation of ESR
spectra. II. |
Saturation transfer spectroscopy of axially symmetric |
spin labels. |
Mol. Phys. |
31, 1703-1715. |
|
Columbus, L., and Hubbell, W.L. (2002). A new spin on protein dynamics. TIBS 27, 288-295. Cone, R.A. (1972). Rotational diffusion of rhodopsin in the visual receptor membrane. Nature
New Biol. 236, 39-43.
Corbett, J.D., Agre, P., Palek, J., and Golan, D.E. (1994). Differential control of band 3 lateral and rotational mobility in intact red cells. J. Clin. Invest. 94, 683-688.
Cornea, R.L., and Thomas, D.D. (1994). Effects of membrane thickness on the molecular dynamics and enzymatic activity of reconstituted Ca-ATPase. Biochemistry 33, 29122920.
Dalton, L.R., B.H. Robinson, L.A. Dalton, and P. Coffey. (1976). Saturation transfer spectroscopy, in Advances in Magnetic Resonance, Volume 8, J.S. Waugh, ed.. Academic Press. New York, pp. 149-249.
Edidin, M. (1974). Rotational and translational diffusion in membranes. Ann. Rev. Biophys. Bioeng. 3,179-201.
Ehrenberg, M., and Rigler, R. (1972). Polarized fluorescence and rotational Brownian motion.
Chem. Phys. Lett. 14, 539-544.
Essman, M., Hideg, K., and Marsh, D. (1992). Conventional and saturation transfer EPR spectroscopy of Na+/K(+)-ATPase modified with different maleimide-nitroxide derivatives. Biochim. Biophys. Acta 1159, 51-59.
Fajer, P., and Marsh, D. (1982). Microwave and modulation field inhomogeneities and the effect of cavity Q in saturation transfer ESR spectra. Dependence on sample size. J. Magn. Reson. 49, 212-224.
Fajer, P., and Marsh, D. (1983). Sensitivity of saturation transfer ESR spectra to anisotropic rotation. Application to membrane systems. J. Magn. Reson. 51, 446-459.