
- •CONTENTS
- •Preface
- •Contributors
- •1 Introduction to Toxicology
- •1.1 Definition and Scope, Relationship to Other Sciences, and History
- •1.1.2 Relationship to Other Sciences
- •1.1.3 A Brief History of Toxicology
- •1.3 Sources of Toxic Compounds
- •1.3.1 Exposure Classes
- •1.3.2 Use Classes
- •1.4 Movement of Toxicants in the Environment
- •Suggested Reading
- •2.1 Introduction
- •2.2 Cell Culture Techniques
- •2.2.1 Suspension Cell Culture
- •2.2.2 Monolayer Cell Culture
- •2.2.3 Indicators of Toxicity in Cultured Cells
- •2.3 Molecular Techniques
- •2.3.1 Molecular Cloning
- •2.3.2 cDNA and Genomic Libraries
- •2.3.3 Northern and Southern Blot Analyses
- •2.3.4 Polymerase Chain Reaction (PCR)
- •2.3.5 Evaluation of Gene Expression, Regulation, and Function
- •2.4 Immunochemical Techniques
- •Suggested Reading
- •3.1 Introduction
- •3.2 General Policies Related to Analytical Laboratories
- •3.2.1 Standard Operating Procedures (SOPs)
- •3.2.2 QA/QC Manuals
- •3.2.3 Procedural Manuals
- •3.2.4 Analytical Methods Files
- •3.2.5 Laboratory Information Management System (LIMS)
- •3.3 Analytical Measurement System
- •3.3.1 Analytical Instrument Calibration
- •3.3.2 Quantitation Approaches and Techniques
- •3.4 Quality Assurance (QA) Procedures
- •3.5 Quality Control (QC) Procedures
- •3.6 Summary
- •Suggested Reading
- •4 Exposure Classes, Toxicants in Air, Water, Soil, Domestic and Occupational Settings
- •4.1 Air Pollutants
- •4.1.1 History
- •4.1.2 Types of Air Pollutants
- •4.1.3 Sources of Air Pollutants
- •4.1.4 Examples of Air Pollutants
- •4.1.5 Environmental Effects
- •4.2 Water and Soil Pollutants
- •4.2.1 Sources of Water and Soil Pollutants
- •4.2.2 Examples of Pollutants
- •4.3 Occupational Toxicants
- •4.3.1 Regulation of Exposure Levels
- •4.3.2 Routes of Exposure
- •4.3.3 Examples of Industrial Toxicants
- •Suggested Reading
- •5 Classes of Toxicants: Use Classes
- •5.1 Introduction
- •5.2 Metals
- •5.2.1 History
- •5.2.2 Common Toxic Mechanisms and Sites of Action
- •5.2.3 Lead
- •5.2.4 Mercury
- •5.2.5 Cadmium
- •5.2.6 Chromium
- •5.2.7 Arsenic
- •5.2.8 Treatment of Metal Poisoning
- •5.3 Agricultural Chemicals (Pesticides)
- •5.3.1 Introduction
- •5.3.3 Organochlorine Insecticides
- •5.3.4 Organophosphorus Insecticides
- •5.3.5 Carbamate Insecticides
- •5.3.6 Botanical Insecticides
- •5.3.7 Pyrethroid Insecticides
- •5.3.8 New Insecticide Classes
- •5.3.9 Herbicides
- •5.3.10 Fungicides
- •5.3.11 Rodenticides
- •5.3.12 Fumigants
- •5.3.13 Conclusions
- •5.4 Food Additives and Contaminants
- •5.5 Toxins
- •5.5.1 History
- •5.5.2 Microbial Toxins
- •5.5.3 Mycotoxins
- •5.5.4 Algal Toxins
- •5.5.5 Plant Toxins
- •5.5.6 Animal Toxins
- •5.6 Solvents
- •5.7 Therapeutic Drugs
- •5.8 Drugs of Abuse
- •5.9 Combustion Products
- •5.10 Cosmetics
- •Suggested Reading
- •6 Absorption and Distribution of Toxicants
- •6.1 Introduction
- •6.2 Cell Membranes
- •6.3 Mechanisms of Transport
- •6.3.1 Passive Diffusion
- •6.4 Physicochemical Properties Relevant to Diffusion
- •6.4.1 Ionization
- •6.5 Routes of Absorption
- •6.5.1 Extent of Absorption
- •6.5.2 Gastrointestinal Absorption
- •6.5.3 Dermal Absorption
- •6.5.4 Respiratory Penetration
- •6.6 Toxicant Distribution
- •6.6.1 Physicochemical Properties and Protein Binding
- •6.7 Toxicokinetics
- •Suggested Reading
- •7 Metabolism of Toxicants
- •7.1 Introduction
- •7.2 Phase I Reactions
- •7.2.4 Nonmicrosomal Oxidations
- •7.2.5 Cooxidation by Cyclooxygenases
- •7.2.6 Reduction Reactions
- •7.2.7 Hydrolysis
- •7.2.8 Epoxide Hydration
- •7.2.9 DDT Dehydrochlorinase
- •7.3 Phase II Reactions
- •7.3.1 Glucuronide Conjugation
- •7.3.2 Glucoside Conjugation
- •7.3.3 Sulfate Conjugation
- •7.3.4 Methyltransferases
- •7.3.7 Acylation
- •7.3.8 Phosphate Conjugation
- •Suggested Reading
- •8 Reactive Metabolites
- •8.1 Introduction
- •8.2 Activation Enzymes
- •8.3 Nature and Stability of Reactive Metabolites
- •8.4 Fate of Reactive Metabolites
- •8.4.1 Binding to Cellular Macromolecules
- •8.4.2 Lipid Peroxidation
- •8.4.3 Trapping and Removal: Role of Glutathione
- •8.5 Factors Affecting Toxicity of Reactive Metabolites
- •8.5.1 Levels of Activating Enzymes
- •8.5.2 Levels of Conjugating Enzymes
- •8.5.3 Levels of Cofactors or Conjugating Chemicals
- •8.6 Examples of Activating Reactions
- •8.6.1 Parathion
- •8.6.2 Vinyl Chloride
- •8.6.3 Methanol
- •8.6.5 Carbon Tetrachloride
- •8.6.8 Acetaminophen
- •8.6.9 Cycasin
- •8.7 Future Developments
- •Suggested Reading
- •9.1 Introduction
- •9.2 Nutritional Effects
- •9.2.1 Protein
- •9.2.2 Carbohydrates
- •9.2.3 Lipids
- •9.2.4 Micronutrients
- •9.2.5 Starvation and Dehydration
- •9.2.6 Nutritional Requirements in Xenobiotic Metabolism
- •9.3 Physiological Effects
- •9.3.1 Development
- •9.3.2 Gender Differences
- •9.3.3 Hormones
- •9.3.4 Pregnancy
- •9.3.5 Disease
- •9.3.6 Diurnal Rhythms
- •9.4 Comparative and Genetic Effects
- •9.4.1 Variations Among Taxonomic Groups
- •9.4.2 Selectivity
- •9.4.3 Genetic Differences
- •9.5 Chemical Effects
- •9.5.1 Inhibition
- •9.5.2 Induction
- •9.5.3 Biphasic Effects: Inhibition and Induction
- •9.6 Environmental Effects
- •9.7 General Summary and Conclusions
- •Suggested Reading
- •10 Elimination of Toxicants
- •10.1 Introduction
- •10.2 Transport
- •10.3 Renal Elimination
- •10.4 Hepatic Elimination
- •10.4.2 Active Transporters of the Bile Canaliculus
- •10.5 Respiratory Elimination
- •10.6 Conclusion
- •Suggested Reading
- •11 Acute Toxicity
- •11.1 Introduction
- •11.2 Acute Exposure and Effect
- •11.3 Dose-response Relationships
- •11.4 Nonconventional Dose-response Relationships
- •11.5 Mechanisms of Acute Toxicity
- •11.5.1 Narcosis
- •11.5.2 Acetylcholinesterase Inhibition
- •11.5.3 Ion Channel Modulators
- •11.5.4 Inhibitors of Cellular Respiration
- •Suggested Reading
- •12 Chemical Carcinogenesis
- •12.1 General Aspects of Cancer
- •12.2 Human Cancer
- •12.2.1 Causes, Incidence, and Mortality Rates of Human Cancer
- •12.2.2 Known Human Carcinogens
- •12.3 Classes of Agents Associated with Carcinogenesis
- •12.3.2 Epigenetic Agents
- •12.4 General Aspects of Chemical Carcinogenesis
- •12.5 Initiation-Promotion Model for Chemical Carcinogenesis
- •12.6 Metabolic Activation of Chemical Carcinogens and DNA Adduct Formation
- •12.7 Oncogenes
- •12.8 Tumor Suppressor Genes
- •12.8.1 Inactivation of Tumor Suppressor Genes
- •12.8.2 p53 Tumor Suppressor Gene
- •12.9 General Aspects of Mutagenicity
- •12.10 Usefulness and Limitations of Mutagenicity Assays for the Identification of Carcinogens
- •Suggested Reading
- •13 Teratogenesis
- •13.1 Introduction
- •13.2 Principles of Teratology
- •13.3 Mammalian Embryology Overview
- •13.4 Critical Periods
- •13.5 Historical Teratogens
- •13.5.1 Thalidomide
- •13.5.2 Accutane (Isotetrinoin)
- •13.5.3 Diethylstilbestrol (DES)
- •13.5.4 Alcohol
- •13.6 Testing Protocols
- •13.6.1 FDA Guidelines for Reproduction Studies for Safety Evaluation of Drugs for Human Use
- •13.6.3 Alternative Test Methods
- •13.7 Conclusions
- •Suggested Reading
- •14 Hepatotoxicity
- •14.1 Introduction
- •14.1.1 Liver Structure
- •14.1.2 Liver Function
- •14.2 Susceptibility of the Liver
- •14.3 Types of Liver Injury
- •14.3.1 Fatty Liver
- •14.3.2 Necrosis
- •14.3.3 Apoptosis
- •14.3.4 Cholestasis
- •14.3.5 Cirrhosis
- •14.3.6 Hepatitis
- •14.3.7 Oxidative Stress
- •14.3.8 Carcinogenesis
- •14.4 Mechanisms of Hepatotoxicity
- •14.5 Examples of Hepatotoxicants
- •14.5.1 Carbon Tetrachloride
- •14.5.2 Ethanol
- •14.5.3 Bromobenzene
- •14.5.4 Acetaminophen
- •14.6 Metabolic Activation of Hepatotoxicants
- •Suggested Reading
- •15 Nephrotoxicity
- •15.1 Introduction
- •15.1.1 Structure of the Renal System
- •15.1.2 Function of the Renal System
- •15.2 Susceptibility of the Renal System
- •15.3 Examples of Nephrotoxicants
- •15.3.1 Metals
- •15.3.2 Aminoglycosides
- •15.3.3 Amphotericin B
- •15.3.4 Chloroform
- •15.3.5 Hexachlorobutadiene
- •Suggested Reading
- •16 Toxicology of the Nervous System
- •16.1 Introduction
- •16.2 The Nervous system
- •16.2.1 The Neuron
- •16.2.2 Neurotransmitters and their Receptors
- •16.2.3 Glial Cells
- •16.3 Toxicant Effects on the Nervous System
- •16.3.1 Structural Effects of Toxicants on Neurons
- •16.3.2 Effects of Toxicants on Other Cells
- •16.4 Neurotoxicity Testing
- •16.4.1 In vivo Tests of Human Exposure
- •16.4.2 In vivo Tests of Animal Exposure
- •16.4.3 In vitro Neurochemical and Histopathological End Points
- •16.5 Summary
- •Suggested Reading
- •17 Endocrine System
- •17.1 Introduction
- •17.2 Endocrine System
- •17.2.1 Nuclear Receptors
- •17.3 Endocrine Disruption
- •17.3.1 Hormone Receptor Agonists
- •17.3.2 Hormone Receptor Antagonists
- •17.3.3 Organizational versus Activational Effects of Endocrine Toxicants
- •17.3.4 Inhibitors of Hormone Synthesis
- •17.3.5 Inducers of Hormone Clearance
- •17.3.6 Hormone Displacement from Binding Proteins
- •17.4 Incidents of Endocrine Toxicity
- •17.4.1 Organizational Toxicity
- •17.4.2 Activational Toxicity
- •17.4.3 Hypothyroidism
- •17.5 Conclusion
- •Suggested Reading
- •18 Respiratory Toxicity
- •18.1 Introduction
- •18.1.1 Anatomy
- •18.1.2 Cell Types
- •18.1.3 Function
- •18.2 Susceptibility of the Respiratory System
- •18.2.1 Nasal
- •18.2.2 Lung
- •18.3 Types of Toxic Response
- •18.3.1 Irritation
- •18.3.2 Cell Necrosis
- •18.3.3 Fibrosis
- •18.3.4 Emphysema
- •18.3.5 Allergic Responses
- •18.3.6 Cancer
- •18.3.7 Mediators of Toxic Responses
- •18.4 Examples of Lung Toxicants Requiring Activation
- •18.4.1 Introduction
- •18.4.2 Monocrotaline
- •18.4.3 Ipomeanol
- •18.4.4 Paraquat
- •18.5 Defense Mechanisms
- •Suggested Reading
- •19 Immunotoxicity
- •19.1 Introduction
- •19.2 The Immune System
- •19.3 Immune Suppression
- •19.4 Classification of Immune-Mediated Injury (Hypersensitivity)
- •19.5 Effects of Chemicals on Allergic Disease
- •19.5.1 Allergic Contact Dermatitis
- •19.5.2 Respiratory Allergens
- •19.5.3 Adjuvants
- •19.6 Emerging Issues: Food Allergies, Autoimmunity, and the Developing Immune System
- •Suggested Reading
- •20 Reproductive System
- •20.1 Introduction
- •20.2 Male Reproductive Physiology
- •20.3 Mechanisms and Targets of Male Reproductive Toxicants
- •20.3.1 General Mechanisms
- •20.3.2 Effects on Germ Cells
- •20.3.3 Effects on Spermatogenesis and Sperm Quality
- •20.3.4 Effects on Sexual Behavior
- •20.3.5 Effects on Endocrine Function
- •20.4 Female Reproductive Physiology
- •20.5 Mechanisms and Targets of Female Reproductive Toxicants
- •20.5.1 Tranquilizers, Narcotics, and Social Drugs
- •20.5.2 Endocrine Disruptors (EDs)
- •20.5.3 Effects on Germ Cells
- •20.5.4 Effects on the Ovaries and Uterus
- •20.5.5 Effects on Sexual Behavior
- •Suggested Reading
- •21 Toxicity Testing
- •21.1 Introduction
- •21.2 Experimental Administration of Toxicants
- •21.2.1 Introduction
- •21.2.2 Routes of Administration
- •21.3 Chemical and Physical Properties
- •21.4 Exposure and Environmental Fate
- •21.5 In vivo Tests
- •21.5.1 Acute and Subchronic Toxicity Tests
- •21.5.2 Chronic Tests
- •21.5.3 Reproductive Toxicity and Teratogenicity
- •21.5.4 Special Tests
- •21.6 In vitro and Other Short-Term Tests
- •21.6.1 Introduction
- •21.6.2 Prokaryote Mutagenicity
- •21.6.3 Eukaryote Mutagenicity
- •21.6.4 DNA Damage and Repair
- •21.6.5 Chromosome Aberrations
- •21.6.6 Mammalian Cell Transformation
- •21.6.7 General Considerations and Testing Sequences
- •21.7 Ecological Effects
- •21.7.1 Laboratory Tests
- •21.7.2 Simulated Field Tests
- •21.7.3 Field Tests
- •21.8 Risk Analysis
- •21.9 The Future of Toxicity Testing
- •Suggested Reading
- •22 Forensic and Clinical Toxicology
- •22.1 Introduction
- •22.2 Foundations of Forensic Toxicology
- •22.3 Courtroom Testimony
- •22.4.1 Documentation Practices
- •22.4.2 Considerations for Forensic Toxicological Analysis
- •22.4.3 Drug Concentrations and Distribution
- •22.5 Laboratory Analyses
- •22.5.1 Colorimetric Screening Tests
- •22.5.2 Thermal Desorption
- •22.5.6 Enzymatic Immunoassay
- •22.6 Analytical Schemes for Toxicant Detection
- •22.7 Clinical Toxicology
- •22.7.1 History Taking
- •22.7.2 Basic Operating Rules in the Treatment of Toxicosis
- •22.7.3 Approaches to Selected Toxicoses
- •Suggested Reading
- •23 Prevention of Toxicity
- •23.1 Introduction
- •23.2 Legislation and Regulation
- •23.2.1 Federal Government
- •23.2.2 State Governments
- •23.2.3 Legislation and Regulation in Other Countries
- •23.3 Prevention in Different Environments
- •23.3.1 Home
- •23.3.2 Workplace
- •23.3.3 Pollution of Air, Water, and Land
- •23.4 Education
- •Suggested Reading
- •24 Human Health Risk Assessment
- •24.1 Introduction
- •24.2 Risk Assessment Methods
- •24.2.2 Exposure Assessment
- •24.2.3 Dose Response and Risk Characterization
- •24.3 Noncancer Risk Assessment
- •24.3.1 Default Uncertainty and Modifying Factors
- •24.3.2 Derivation of Developmental Toxicant RfD
- •24.3.3 Determination of RfD and RfC of Naphthalene with the NOAEL Approach
- •24.3.4 Benchmark Dose Approach
- •24.3.5 Determination of BMD and BMDL for ETU
- •24.3.6 Quantifying Risk for Noncarcinogenic Effects: Hazard Quotient
- •24.3.7 Chemical Mixtures
- •24.4 Cancer Risk Assessment
- •24.5 PBPK Modeling
- •Suggested Reading
- •25 Analytical Methods in Toxicology
- •25.1 Introduction
- •25.2 Chemical and Physical Methods
- •25.2.1 Sampling
- •25.2.2 Experimental Studies
- •25.2.3 Forensic Studies
- •25.2.4 Sample Preparation
- •25.2.6 Spectroscopy
- •25.2.7 Other Analytical Methods
- •Suggested Reading
- •26 Basics of Environmental Toxicology
- •26.1 Introduction
- •26.2 Environmental Persistence
- •26.2.1 Abiotic Degradation
- •26.2.2 Biotic Degradation
- •26.2.3 Nondegradative Elimination Processes
- •26.3 Bioaccumulation
- •26.4 Toxicity
- •26.4.1 Acute Toxicity
- •26.4.2 Mechanisms of Acute Toxicity
- •26.4.3 Chronic Toxicity
- •26.4.5 Abiotic and Biotic Interactions
- •26.5 Conclusion
- •Suggested Reading
- •27.1 Introduction
- •27.2 Sources of Toxicants to the Environment
- •27.3 Transport Processes
- •27.3.1 Advection
- •27.3.2 Diffusion
- •27.4 Equilibrium Partitioning
- •27.5 Transformation Processes
- •27.5.1 Reversible Reactions
- •27.5.2 Irreversible Reactions
- •27.6 Environmental Fate Models
- •Suggested Reading
- •28 Environmental Risk Assessment
- •28.1 Introduction
- •28.2 Formulating the Problem
- •28.2.1 Selecting Assessment End Points
- •28.2.2 Developing Conceptual Models
- •28.2.3 Selecting Measures
- •28.3 Analyzing Exposure and Effects Information
- •28.3.1 Characterizing Exposure
- •28.3.2 Characterizing Ecological Effects
- •28.4 Characterizing Risk
- •28.4.1 Estimating Risk
- •28.4.2 Describing Risk
- •28.5 Managing Risk
- •Suggested Reading
- •29 Future Considerations for Environmental and Human Health
- •29.1 Introduction
- •29.2 Risk Management
- •29.3 Risk Assessment
- •29.4 Hazard and Exposure Assessment
- •29.5 In vivo Toxicity
- •29.6 In vitro Toxicity
- •29.7 Biochemical and Molecular Toxicology
- •29.8 Development of Selective Toxicants
- •Glossary
- •Index
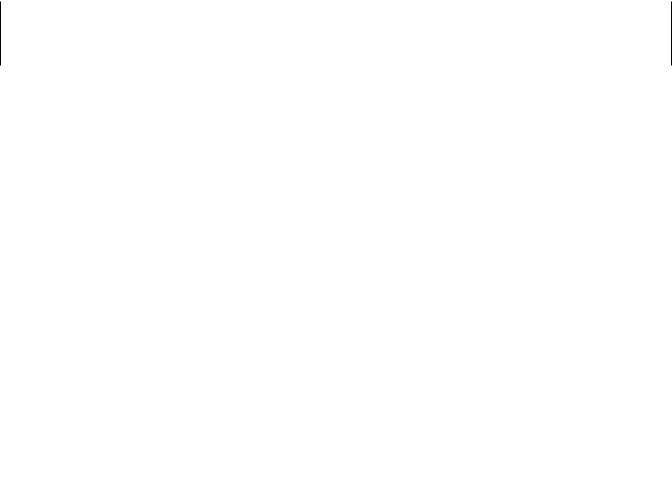
248 CHEMICAL CARCINOGENESIS
colon cancers, 50% of breast and lung cancers, and 97% of primary melanomas. In addition to point mutations, allelic loss, rearrangements, and deletions of p53 occur in human tumors. p53 is a transcription factor and participates in many cellular functions, including cell cycle regulation, DNA repair, and apoptosis. The p53 protein is composed of 393 amino acids, and single missense mutations can inactivate the p53. Unlike ras genes, which have a few mutational codons that result in its activation, the p53 protein can be inactivated by hundreds of different single-point mutations in p53. It has been proposed that the mutation spectrum of p53 in human cancer can aid in the identification of the specific carcinogen that is responsible for the genetic damage; that is to say, different carcinogens cause different characteristic mutations in p53. Some of the mutations in p53 reflect endogenous oxidative damage, while others such as the mutational spectrum in p53 in hepatocellular carcinomas from individuals exposed to aflatoxin demonstrate a mutation spectrum characteristic aflatoxin. In sun-exposed areas where skin tumors develop, the mutations found in p53 in these tumors are characteristic of UV light induced pyrimidine dimers, and finally the mutation spectrum induced by (+)-benzo[a]pyrene-7,8-diol-9,10-epoxide-2 in cells in culture is similar to the mutational spectrum in p53 in lung tumors form cigarette smokers. Thus certain carcinogens produce a molecular signature that may provide important information in understanding the etiology of tumor development.
p53 has been termed the “guardian of genome” because it controls a G1/S checkpoint, regulates DNA repair, and apoptosis. DNA damage results in the activation of p53 function and p53 prevents cells with damaged DNA from entering the S-phase of the cell cycle until the DNA damage is repaired. If the DNA damage is severe, p53 can cause the cell to undergo apoptosis. Mutation of p53 disrupts these functions leading to the accumulation of mutations as cells enter S-phase with damaged DNA (mutator phenotype, genetic instability) and further development of malignant clones.
12.9GENERAL ASPECTS OF MUTAGENICITY
Mutagens are chemical and physical agents that are capable of producing a mutation. Mutagens include agents such as radiation, chemotherapeutic agents, and many carcinogens. A mutation is a permanent alteration in the genetic information (DNA) of the cell. DNA-damaging agents/mutagens can produce (1) point mutations involving single base pair substitutions that can result in amino acid substitutions in the encoded protein and frame-shift mutations involving the loss or gain of one or two base pairs, resulting in an altered reading frame and gross alterations in the encoded protein,
(2) chromosome aberrations including gross chromosomal rearrangement such as deletions, duplications, inversions, and translocations, and (3) aneuploidy and polyploidy, which involve the gain or loss of one or more chromosomes. Point mutations are classified as missense or nonsense mutations. A missense mutation produces an altered protein in which an incorrect amino acid has been substituted for the correct amino acid. A nonsense mutation is an alteration that produces a stop codon and results in a truncated protein. A point mutation can also be characterized based on the mutageninduced substitution of one base for another within the DNA. When a point mutation produces a substitution of a purine for another purine (i.e., guanine for adenine) or a pyrimidine for another pyrimidine (i.e., thymine for cytosine), the mutation is referred
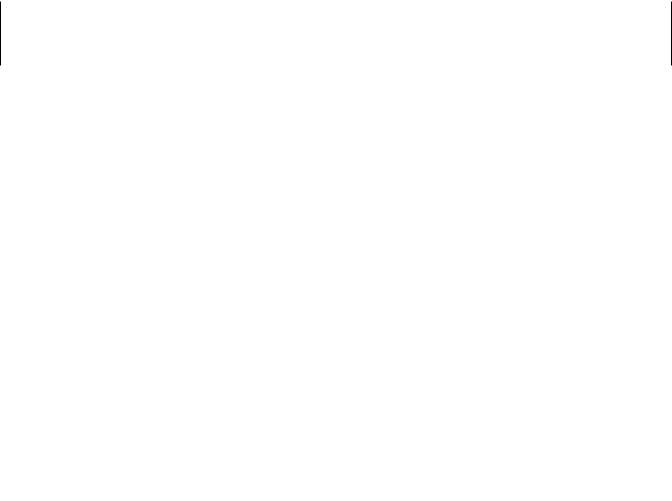
USEFULNESS AND LIMITATIONS OF MUTAGENICITY ASSAYS |
249 |
as a transition. If a purine is substituted for a pyrimidine, and vice versa (i.e., thymine for adenine or guanine for cytosine), the mutation is referred to as a transversion.
12.10 USEFULNESS AND LIMITATIONS OF MUTAGENICITY ASSAYS FOR THE IDENTIFICATION OF CARCINOGENS
As mentioned earlier in this chapter, the two-year rodent carcinogenesis bioassay is considered the “gold standard” and is utilized to determine whether a test compound has carcinogenic potential. Identification and classification of potential human carcinogens through the two-year rodent carcinogenesis bioassay is complicated by species differences, use of high doses (MTD, maximum tolerated dose), the short life span of the rodents, sample size, and the need to extrapolate from high to low doses for human risk assessment. In addition the two-year rodent bioassay to is costly to conduct (>2 million dollars) and takes two to four years before complete results can be obtained. Since many carcinogens are mutagens, short-term test systems to evaluate the mutagenicity or genetic toxicity of compounds were developed with the idea that these tests could be used to quickly and inexpensively detect/identify chemical carcinogens. Short-term genotoxicity/mutagenicity assays were developed in a variety of organisms including bacteria, yeast, Drosophila, and human and rodent cells. These mutagenic assays or short-term genotoxicity tests directly or indirectly measure point mutations, frame-shift mutations, chromosomal damage, DNA damage and repair, and cell transformation.
In the 1970s it was reported that mutagenicity could predict rodent carcinogenicity 90% of the time. However, after extensive evaluation, it is now considered that mutagenicity can predict rodent carcinogenicity approximately 60% of the time. For certain classes of carcinogens such as the polycyclic aromatic hydrocarbons, short-term mutagenicity tests are generally highly accurate at predicting rodent carcinogenicity. For other classes of carcinogens such as the halogenated hydrocarbons, short-term genotoxicity tests often fail to detect these rodent carcinogens. Many of these halogenated hydrocarbons probably function through an epigenetic mechanism/tumor promoting mechanism.
In an important study published in 1987 by Tennant et al. (Science 236, pp. 933–941) 73 chemicals previously tested in the rodent two-year carcinogenesis bioassay were examined in four widely used short-term tests for genetic toxicity. The short-term assays measured mutagenesis in the Salmonella assay (Ames Assay) and mouse lymphoma assay, and chromosome aberrations and sister chromatid exchanges in Chinese hamster ovary cells. The concordance (% agreement between short-term genotoxicity test and rodent bioassay results) of each assay with the rodent bioassay data was approximately 60%. Within the limits of the study there was no evidence of complementarity among the four tests, and no battery of tests constructed from these assays improved substantially on the overall performance of the Salmonella assay. When interpreting the results of short-term test for genetic toxicity assays, it is important to consider (1) the structure and physical properties of the test compound,
(2) the 60% concordance between the short-term test for genetic toxicity and rodent carcinogenicity, (3) epigenetic versus genetic mechanisms of carcinogenesis, and
(4) the existence noncarcinogenic mutagens. It is |
also important to keep in mind |
that there is accumulating evidence that some |
compounds that are negative in |
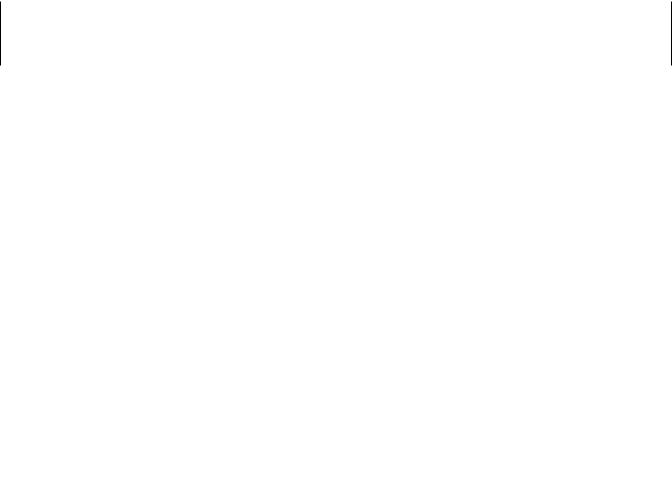
250 CHEMICAL CARCINOGENESIS
short-term tests for mutagenicity can induce oxidative DNA damage in vivo through the direct or indirect production of reactive oxygen species. These compounds are in vivo mutagens but are negative in the short-term test of genetic toxicity.
Several bacterial and mammalian short-term tests for genetic toxicity as well as their biochemical and genetic rationale are described in Chapter 21 on toxicity testing. They include the salmonella assay, the Chinese hamster ovary cell/hypoxanthine-guanine phosphoribosyl transferase assay, the mouse lymphoma assay, the mammalian transformation assay, sister chromatid exchange, and the chromosome aberration assay.
SUGGESTED READING
10 th Report on Carcinogens, US Department of Health and Human Services, Public Health Service, National Toxicology Program. http : //ehp.niehs.nih.gov/roc.toc10.html
Cancer Facts and Figures, American Cancer Society. www.cancer.org
International Agency for Research on Cancer. www.iarc.fr
Pitot, H. C., and Y. P. Dragan. Chemical Carcinogenesis. In Casarett and Doull’s Toxicology: The Basic Science of Poisons, 6th ed., C. D. Klaassen, ed. New York: McGraw Hill, 2001, pp 241–319.
Smart, R. C., and J. K. Akunda. Carcinogenesis. In An Introduction to Biochemical Toxicology, E. Hodgson and R. C. Smart, eds. New York: Wiley, 2001, pp 343–396.
Tennant, R. W., et al. Prediction of chemical carcinogenicity in rodents from in vitro genetic toxicity tests. Science 236: 933–941, 1987.
- #15.08.20134.04 Mб17Hastie T., Tibshirani R., Friedman J. - The Elements of Statistical Learning Data Mining, Inference and Prediction (2002)(en).djvu
- #
- #
- #
- #
- #
- #
- #
- #15.08.201315.44 Mб26Hudlicky M, Pavlath A.E. (eds.) - Chemistry of Organic Fluorine Compounds 2[c] A critical Review (1995)(en).djvu
- #
- #