
Patterson, Bailey - Solid State Physics Introduction to theory
.pdf
8.5 The Theory of Superconductivity (A) |
497 |
|
|
and G can be put in the form |
|
||
G = ∑k (εk − Ek + k |
|
|
(8.198) |
bk ) . |
|||
Note by Fig. 8.20 and (8.193) how Ek predicts a gap, for clearly Ek ≥ |
0. Continu- |
||
ing |
|
||
bk = −vkukαk†αk + uk vk βk βk† − vk2αk† βk† + uk2βkαk . |
(8.199) |
¯ |
|
|
|
|
|
|
|
|
|
|
|
|
|
But bk involves only diagonal terms, so using an appropriate anticommutation re- |
|||||||||||||
lation |
|
|
|
|
|
|
|
|
|
|
|
||
|
bk = uk vk 1− |
|
|
− |
|
, |
|
||||||
|
αk†αk |
βk†βk |
(8.200) |
||||||||||
so |
|
|
|
|
|
|
|
|
|
|
|
||
|
|
|
|
|
|
|
(8.201) |
||||||
|
|
bk = ukvk (1− 2nk ) , |
|||||||||||
where |
|
|
|
|
|
|
|
|
|
|
|
||
|
|
nk |
= |
|
1 |
|
|
= f (Ek ) . |
(8.202) |
||||
|
|
e |
βEk +1 |
||||||||||
|
|
|
|
|
|
|
|
|
|
f(Ek) is of course the Fermi function but it looks strange without the chemical potential. This is because α†, β† do not change the particle number. See Marder [8.22]. Therefore,
k = −∑Vk,k′ |
|
|
|
|
|
|
|
|
|
|
|
|
|
|
|
|
|
|
|
|
|||
bk′ |
|
|
|
|
|
|
|
|
|
|
|
|
|
|
|
|
|
||||||
= −∑Vk,k′uk′vk′[1− 2 f (Ek′)] |
(8.203) |
||||||||||||||||||||||
= −∑Vk,k′ |
|
|
|
k′ |
[1− 2 f (Ek′)]. |
|
|||||||||||||||||
|
|
|
|
|
|
||||||||||||||||||
k′ |
|
|
|
2Ek′ |
|
|
|
|
|
|
|
|
|
||||||||||
Now assume that (not using = 1) |
|
|
|
|
|
|
|
|
|
|
|
|
|
|
|
|
|
|
|
|
|
||
k′ = |
|
|
when |
|
|
εk |
|
< |
ωD , |
(8.204) |
|||||||||||||
|
|
|
|
|
|||||||||||||||||||
Vk,k′ = −V |
when |
|
|
|
εk |
|
< |
ωD , |
(8.205) |
||||||||||||||
|
|
|
|
||||||||||||||||||||
k = 0 |
|
when |
|
εk |
|
|
> |
ωD , |
(8.206) |
||||||||||||||
|
|
|
|||||||||||||||||||||
and |
|
|
|
|
|
|
|
|
|
|
|
|
|
|
|
|
|
|
|
|
|
|
|
Vk,k′ = 0 |
when |
|
|
εk |
|
|
> |
ωD , |
(8.207) |
||||||||||||||
|
|
|
|||||||||||||||||||||
where ωD is the Debye frequency (see (8.138)) So, |
|
|
|
||||||||||||||||||||
=V ∑ |
|ε |
|
|< ωD |
|
|
[1− 2 f (Ek′)] |
. |
(8.208) |
|||||||||||||||
|
|
|
|
||||||||||||||||||||
|
k′ |
|
|
|
|
|
|
2Ek |
′ |
|
|||||||||||||
|
|
|
|
|
|
|
|
|
|
|
|
|

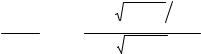
|
|
|
8.5 The Theory of Superconductivity |
(A) 499 |
|||||||||
|
|
|
|
||||||||||
in the weak coupling limit (when << |
ωD). Thus, in the weak coupling limit, we |
||||||||||||
obtain |
|
|
|
|
|
|
|
|
|
|
|
|
|
|
|
|
|
|
|
|
|
1 |
|
|
|
|
|
|
2 ω |
D |
exp |
− |
|
|
|
|
|
. |
|
(8.214) |
|
|
|
|
|
|
|
|
|||||||
|
|
|
|
|
|
|
|
|
|
|
|
|
|
|
|
|
|
|
|
|
N (0)V |
|
|
||||
From (8.210) by similar reasoning |
|
|
|
|
|
|
|
|
|
|
|
||
|
|
|
|
|
ε |
2 |
+ |
2 |
|
|
|
|
|
1 |
|
ωD |
tanh |
|
|
|
2kT |
|
|
||||
= ∫ |
|
|
|
|
|
|
|
|
|
dε , |
(8.215) |
||
N (0)V |
|
|
|
|
2 |
|
2 |
|
|
||||
0 |
|
|
|
ε |
+ |
|
|
|
|
||||
|
|
|
|
|
|
|
|
|
|
|
|
where, again, N(0) is the density of states at the Fermi energy.
For T greater than some critical temperature there are no solutions for , i.e. the energy gap no longer exists. We can determine Tc by using the fact that at T = Tc,
= 0. This says that
1 |
= ∫ |
ωD |
tanh(ε / 2kTc ) |
dε . |
(8.216) |
N (0)V |
|
||||
0 |
|
ε |
|
In the weak coupling approximation, when N(0)V << 1, we obtain from (8.216) that
kTc =1.14 ωD exp(−1/ N (0)V ) . |
(8.217) |
Equation (8.217) is a very important equation. It depends on three material properties:
a)The Debye frequency ωD
b)V that measures the strength of the electron–phonon coupling and
c)N(0) that measures the number of electrons available at the Fermi energy.
Note that typically ωD (m)–1/2, where m is the mass of atoms. This leads directly to the isotope effect. Note also the energy gap Eg = 2 (0) at absolute zero.
We can combine this result with our result for the energy gap parameter in the ground state to derive a relation between the energy gap at absolute zero and the critical superconducting transition temperature with no magnetic field. By (8.217) and (8.214), we have that
(0) = 2 |
ω |
D |
exp(−1/ N (0)V ) = |
|
2 |
kT , |
(8.218) |
|
|
||||||
|
|
|
1.14 |
c |
|
||
|
|
|
|
|
|
||
or |
|
|
|
|
|
|
|
|
|
2 |
(0) = 3.52kTc . |
|
|
|
(8.219) |
Note that our expression for |
(0) and Tc both involve the factor exp(−1/N(0)V); that |
is, a power series (in V) expansion for both (0) and Tc have an essential singu larity in V. We could not have obtained reasonable results if we had tried ordinary


8.7 Heavy-Electron Superconductors (EE, MS, MET) 501
The calculation includes the self-energy terms. The lowest-order correction to self-energy for electrons due to phonons is indicated in Fig. 8.21. The BCS theory with the extension of Eliashberg and McMillan has been very successful for many superconductors.
A nice reference to consult is Mattuck [8.23 pp. 267-272].
8.6 Magnesium Diboride (EE, MS, MET)
For a review of the new superconductor magnesium diboride, see, e.g., Physics Today, March 2003, p. 34ff. The discovery of the superconductor MgB2, with a transition temperature of 39 K, was announced by Akimitsu in early 2001. At first sight this might not appear to be a particularly interesting discovery, compared to that of the high-temperature superconductors, but MgB2 has several interesting properties:
1.It appears to be a conventional BCS superconductor with electron–phonon coupling driving the formation of pairs. It shows a strong isotope effect.
2.It does not appear to have the difficulty that the high-Tc cuprate ceramics have of having grain boundaries that inhibit current.
3.It is a widely available material that comes right off the shelf.
4.MgB2 is an intermetallic (two metals forming a crystal structure at a welldefined stoichiometry) compound with a transition temperature near double that of Nb3Ge.
Possibly, the transition temperature can be driven higher by tailoring the properties of magnesium diboride. At this writing, several groups are working intensely on this material, with several interesting results including the fact that it has two superconducting gaps arising from two weakly interacting bands.
8.7 Heavy-Electron Superconductors (EE, MS, MET)
UBe13 (Tc = 0.85 K), CeCu2Si2 (Tc = 0.65 K), and UPt3 (Tc = 0.54 K) are heavyelectron superconductors. They are characterized by having large low-temperature specific heats due to effective mass being two or three orders of magnitude larger than in normal metals (because of f band electrons). Heavy-electron superconductors do not appear to have a singlet state s-wave pairing, but perhaps can be characterized as d-wave pairing or p-wave pairing (d and p referring to orbital symmetry). It is also questionable whether the pairing is due to the exchange of virtual phonons—it may be due, e.g., to the exchange of virtual magnons. See, e.g., Burns [8.9 p51]. We have already mentioned these in Sect. 5.7.


8.8 High-Temperature Superconductors (EE, MS, MET) 503
mechanism necessary to produce the pair is still not clear as of this writing. It could be due to magnetic interactions or there may be new physics. See, e.g., Burns [8.9].
Table 8.1. Superconductors and their transition temperatures
Selected elements* |
Transition temperature Tc (K) |
|
Al |
1 |
.17 |
Hg |
4 |
.15 |
Nb |
9 |
.25 |
Sn |
3 |
.72 |
Pb |
7 |
.2 |
|
|
|
Selected compounds* |
|
|
|
|
|
Nb3Ge |
23 |
.2 |
Nb3Sn |
18. |
|
Nb3Au |
10 |
.8 |
NbSe2 |
7 |
.2 |
MgB2** |
39 |
|
Copper oxide (HTS)* |
|
|
|
|
|
Bi2Sr2Ca2Cu3O10 |
~110 |
|
YBa2Cu3O7 |
~92 |
|
Tl2Ba2Ca3Cu4O11 |
~122 |
|
|
|
|
Heavy fermion* |
|
|
|
|
|
UBe13 |
0 |
.85 |
CeCu2Si2 |
0 |
.65 |
UPt3 |
0 |
.54 |
Fullerenes*** |
|
|
|
|
|
K3C60 |
19 |
.2 |
RbCs2C60 |
33 |
|
*Reprinted from Burns G, High Temperature Superconductivity Table 2-1 p. 8 and Table 3-1 p. 57, Academic Press, Copyright 1992, with permission from Elsevier. On p. 52 Burns also briefly discusses organic superconductors.
**Canfield PC and Crabtree GW, Physics Today 56(3), 34 (2003).
***Huffmann DR, “Solid C60,” Physics Today 41(11), 22 (1991).
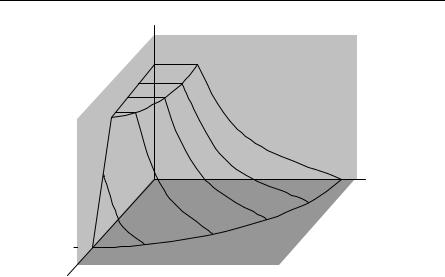

8.9 Summary Comments on Superconductivity (B) 505
5.In zero magnetic fields, for weak superconductors, superconductivity occurs at the transition temperature:
k |
T |
1.14 ω |
D |
exp(−1/ N V ) , |
(8.220) |
|
B c |
|
0 0 |
|
where N0 is half the density of single-electron states, V0 is the effective interaction between electron pairs near the Fermi surface, and ωD θD, where θD is the Debye temperature.
6. The energy gap (2 ) is determined by (weak coupling):
(0) 2 ωD exp(−1/ N0V0 ) =1.76kTc |
(8.221) |
|||||||||||
|
|
|
|
|
T 1/ 2 |
|
|
. |
(8.222) |
|||
(T ) |
|
|
|
|
|
|
T ≤ Tc |
|||||
(0) 1 |
− T |
|
; |
|
|
|||||||
|
|
|
|
|
c |
|
|
|
|
|
||
7. The critical field is fairly close to the empirical law (for weak coupling): |
||||||||||||
|
H |
c |
(T ) |
|
|
T |
|
2 |
|
|
||
|
|
|
|
|
|
|
|
. |
|
(8.223) |
||
|
H |
c |
(0) |
|
≈1− T |
|
|
|
|
|||
|
|
|
|
|
|
c |
|
|
|
|
8.The coherent motion of the electrons results in a resistanceless flow because a small perturbation cannot disturb one pair of electrons without disturbing all of them. Thus, even a small energy gap can inhibit scattering.
9.The central properties of superconductors are the penetration depth λ (of magnetic fields) and the coherence length ξ (or “size” of Cooper pairs). Small λ/ξ ratios lead to type I superconductors, and large λ/ξ ratios lead to type II behavior. ξ can be decreased by alloying.
10.The Ginzburg–Landau theory is used for superconductors in a magnetic field where one has inhomogeneities in spatial behavior.
11.We should also mention that one way to think about the superconducting transition is a Bose–Einstein condensation, as modified by their interaction, of bosonic Cooper pairs.
12.See the comment on spontaneously broken symmetry in the chapter on magnetism. Superconductivity can be viewed as a broken symmetry.
13.In the paired electrons of superconductivity, in s and d waves, the spins are antiparallel, and so one understands why ferromagnetism and superconductivity don’t appear to coexist, at least normally. However, even p-wave superconductors (e.g. Strontium Ruthenate) with parallel spins the magnetic fields are commonly expelled in the superconducting state. Recently, however, two materials have been discovered in which ferromagnetism and superconductivity coexist.
They are UGe2 (under pressure) and ZrZn2 (at ambient pressure). One idea is that these two materials are p-wave superconductors. The issues about these materials are far from settled, however. See Physics Today, p. 16, Sept. 2001.
14.Also, high-Tc (over 100 K) superconductors have been discovered and much work remains to understand them.
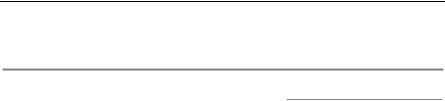