
- •Preface
- •Acronyms
- •Introduction
- •Background and objectives
- •Content, format and presentation
- •Radioactive waste management in context
- •Waste sources and classification
- •Introduction
- •Radioactive waste
- •Waste classification
- •Origins of radioactive waste
- •Nuclear fuel cycle
- •Mining
- •Fuel production
- •Reactor operation
- •Reprocessing
- •Reactor decommissioning
- •Medicine, industry and research
- •Medicine
- •Industry
- •Research
- •Military wastes
- •Conditioning of radioactive wastes
- •Treatment
- •Compaction
- •Incineration
- •Conditioning
- •Cementation
- •Bituminisation
- •Resin
- •Vitrification
- •Spent fuel
- •Process qualification/product quality
- •Volumes of waste
- •Inventories
- •Inventory types
- •Types of data recorded
- •Radiological data
- •Chemical data
- •Physical data
- •Secondary data
- •Radionuclides occurring in the nuclear fuel cycle
- •Simplifying the number of waste types
- •Radionuclide inventory priorities
- •Material priorities
- •Inventory evolution
- •Assumptions
- •Errors
- •Uncertainties
- •Conclusions
- •Acknowledgements
- •References
- •Development of geological disposal concepts
- •Introduction
- •Historical evolution of geological disposal concepts
- •Geological disposal
- •Definitions and comparison with near-surface disposal
- •Development of geological disposal concepts
- •Roles of the geosphere in disposal options
- •Physical stability
- •Hydrogeology
- •Geochemistry
- •Overview
- •Alternatives to geological disposal
- •Introduction
- •Politically blocked options: sub-seabed and Antarctic icecap disposal
- •Sea dumping and sub-seabed disposal
- •Antarctic icesheet disposal
- •Technically impractical options; partitioning and transmutation, space disposal and icesheet disposal
- •Partitioning and Transmutation
- •Space disposal
- •Icesheets and permafrost
- •Non-options; long-term surface storage
- •Alternatives to conventional repositories
- •Introduction
- •Alternative geological disposal concepts
- •Utilising existing underground facilities
- •Extended storage options (CARE)
- •Injection into deep aquifers and caverns
- •Deep boreholes
- •Rock melting
- •The international option: technical aspects
- •Alternative concepts: fitting the management option to future boundary conditions
- •Conclusions
- •References
- •Site selection and characterisation
- •Introduction
- •Prescriptive/geologically led
- •Sophisticated/advocacy led
- •Pragmatic/technically led
- •Centralised/geologically led
- •Conclusions to be drawn
- •Lessons to be learned (see Table 4.2)
- •Site characterisation
- •Can we define the natural environment sufficiently thoroughly?
- •Sedimentary environments
- •Hydrogeology
- •The regional hydrogeological model
- •More local hydrogeological model(s)
- •Crystalline rock environments
- •Lithology and structure
- •Hydrogeology
- •Hydrogeochemistry
- •Any geological environment
- •References
- •Repository design
- •Introduction: general framework of the design process
- •Identification of design requirements/constraints
- •Concept development
- •Major components of the disposal system and safety functions
- •A structured approach for concept development
- •Detailed design/specifications of subsystems
- •Near-field processes and design issues
- •Design approach and methodologies
- •Design confirmation and demonstration
- •Interaction with PA/SA
- •Demonstration and QA
- •Repository management
- •Future perspectives
- •References
- •Assessment of the safety and performance of a radioactive waste repository
- •Introduction
- •The role of SA and the safety case in decision-making
- •SA tasks
- •System description
- •Identification of scenarios and cases for analysis
- •Consequence analysis
- •Timescales for evaluation
- •Constructing and presenting a safety case
- •References
- •Repository implementation
- •Legal and regulatory framework; organisational structures
- •Waste management strategies
- •The need for a clear policy and strategy
- •Timetables vary widely
- •Activities in development of a geological repository
- •Concept development
- •Siting
- •Repository design
- •Licensing
- •Construction
- •Operation
- •Monitoring
- •Research and development
- •The staging process
- •Attributes of adaptive staging
- •The decision-making process
- •Status of geological disposal programmes
- •Overview
- •Status of geological disposal projects in selected countries
- •International repositories
- •Costs and financing
- •Cost estimates
- •Financing
- •Conclusions
- •Acknowledgements
- •References
- •Research and development infrastructure
- •Introduction: Management of research and development
- •Drivers for research and development
- •Organisation of R&D
- •R&D in specialised (nuclear) facilities
- •Introduction
- •Inventory
- •Release of radionuclides from waste forms
- •Solubility and sorption
- •Waste form dissolution
- •Colloids
- •Organic degradation products
- •Gas generation
- •Conventional R&D
- •Engineered barriers
- •Corrosion
- •Buffer and backfill materials
- •Container fabrication
- •Natural barriers
- •Geochemistry and groundwater flow
- •Gas transport and two-phase flow
- •Biosphere
- •Radionuclide concentration and dispersion in the biosphere
- •Climate change
- •Landscape change
- •Underground rock laboratories
- •URLs in sediments
- •Nature’s laboratories: studies of the natural environment
- •General
- •Corrosion
- •Cement
- •Clay materials
- •Degradation of organic materials
- •Glass corrosion
- •Radionuclide migration
- •Model and database development
- •Conclusions
- •References
- •Building confidence in the safe disposal of radioactive waste
- •Growing nuclear concerns
- •Communication systems in waste management programmes
- •The Swiss programme
- •The Japanese programme
- •Examples of communication styles in other countries
- •Finland
- •Sweden
- •France
- •United Kingdom
- •Comparisons between communication styles in Finland, France, Sweden and the United Kingdom
- •Lessons for the future
- •What is the way forward?
- •Acknowledgements
- •References
- •A look to the future
- •Introduction
- •Current trends in repository programmes
- •Priorities for future efforts
- •Waste characterisation
- •Operational safety
- •Emplacement technologies
- •Knowledge management
- •Alternative designs and optimisation processes
- •Materials technology
- •Novel construction/immobilisation materials: the example of low pH cement
- •Future SA code development
- •Implications for environmental protection: disposal of other wastes
- •Conclusions
- •References
- •Index
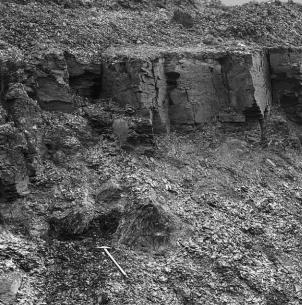
96 |
T. McEwen |
Fig. 4.7. The Opalinus Clay in the Siblingen clay pit, Switzerland. Note the marked planar jointing (image courtesy of Nagra).
provides access to the rock mass on a larger scale than is possible in boreholes and allows better correlations to be developed between boreholes. Where exposure is limited, maximum use should therefore be made of man-made structures such as quarries, brick pits, etc., combined, where appropriate, with other survey methods, such as aerial geophysical techniques, geochemical mapping, multiple shallow boreholes, etc.
Where possible, access to the underground should be sought in tunnels, mines, etc. Such structures, even if they are at considerably shallower depths than is expected for a repository, can provide useful information on the larger scale on geological structures, groundwater flow, etc., especially if records of tunnelling are examined, as is indicated above. Great care has to be taken, however, when the only sources of data are shallow tunnels and boreholes (e.g., JNC, 2000) as this can clearly produce a biased database which may not reflect the rock properties at repository depth (cf. the example above of the Opalinus Clay). This can lead to very pessimistic assumptions on the likely qualities of the repository host rock, possibly leading to ‘‘over-engineering’’ of the EBS in later assessments.
4.2.2.1. Hydrogeology
To understand the groundwater flow system in and around the site under investigation, it will be necessary to study the hydraulic properties of the formations and the distribution of hydraulic heads, possibly over a large area. The geological investigations will provide the structure of the site and the lithological distributions, from which a hydrogeological model, or models, can begin to be developed (e.g., Fig. 4.8, which illustrates the link between the stratigraphy, lithology and the way in which the rock types are represented in the groundwater flow models). It is likely that this modelling will be carried out on different scales, from the regional to the local or site scale.

Site selection and characterisation |
97 |
W
Campine Complex Sand, Clay
Quaternary
Merksplas & Brasschaat: Sand
Lillo Formation: Sand, Clay
Pliocene
Kattendijk Formation: Sand
Diest Formation: Sand
Miocene
Berchem Formation: Sand
Eigenbilzen: Sand
Boom Formation: Clay
Oligocene
Zelzate Formation:
Sand, Clay
Maldegem Formation:
Sand, Clay
Senne Group: Sand
Eocene
leper Group: Sand, Clay
Landen Group: Sand, Clay
Paleocene
Heers Formation: Marl, Sand
Cretaceous |
Maastrichtian: Tuffeau, Chalk |
Aquifer layer
Aquifer layer of high hydraulic conductivity Semi-permeable and impermeable layers
Aquifer layer of secondary permeability
Quaternary Aquifer
Pliocene
Aquifer
Lillo
Aquitard
Neogene
Miocene
Aquifer Aquifer
Boom Aquitard
Under-Rupelian Aquifer
Asse |
Aq. |
|
|
|
|
|
|
Lede-Br |
|
|
|
|
ussel |
|
|
|
|
Aquifer |
|
Ypresian Aquitard |
|||
|
|
|
r |
|
Landen |
Aquife |
|
|
|
Aquifer
Poor-quality aquifer
Aquitard
E
Meuse Terraces: Gravel, Sand |
Quaternary |
|
Mol: Sand |
|
|
|
|
|
Poederlee: Sand |
|
|
Kasterlee: Sand |
Pliocene |
|
|
||
Diest Formation: Sand |
|
|
|
|
Miocene |
Bolderberg Formation: Sand |
|
|
Voort Formation: Sand |
|
|
Eigenbilzen: Sand |
|
|
Boom Formation: Clay |
|
|
K.-N.C.: Clay, Sand |
Bilzen |
Oligocene |
|
||
Berg: Sand |
Formation |
|
|
|
|
Borgloon Formation: Sand, Clay |
|
|
Sint-Huilbrechts-Hern Formation: |
|
|
Sand, Clay |
|
|
Landen Group: Sand, Clay |
|
|
Heers Formation: Marl, Sand |
Palaeocene |
|
Haspengouw Group: Sand, Clay |
|
|
Maastrichtian: Tuffeau, Chalk |
Cretaceous |
K.-N.C.: Kerniel, Nucula Compta
Fig. 4.8. Schematic succession (stratigraphy, lithology and representation for the hydrogeological modelling) of the aquifers and aquitards of the Campine Basin of northern Belgium from the Cretaceous to the Quaternary, showing the Boom Aquitard (ONDRAF, 2001a,b).
Hydraulic testing in low-permeability clays can be difficult and is prone to errors if there is insufficient understanding of how the results of the testing can be influenced by the interaction between the test itself and the clay. Artefacts can be introduced by the various physico-chemical processes that can take place in the clay surrounding the test section, for example swelling of the clay minerals or borehole convergence, both of which could be misinterpreted as an enhanced flow towards the test section and, therefore, as enhanced conductivity. The results of such testing in the Opalinus Clay, which displays a uniformly low permeability and in which anomalous overpressures are present, are shown in Fig. 4.9. Testing in more permeable sediments, such as sandstones and limestones, does not normally suffer from such problems – so that it is the testing of the repository host formation that may prove the most problematic.
4.2.2.2. The regional hydrogeological model
A regional hydrogeological model will encompass the entire hydrogeological system including the host formation and may cover an extensive area; in the case of the Boom Clay this was 7000 m2 (Fig. 4.10). Its purpose is to help understand the hydrogeological system by studying the flow of groundwater in each of the aquifer units that may

98 |
T. McEwen |
Fig. 4.9. Hydraulic testing in the Benken Borehole, northern Switzerland, showing low hydraulic conductivity and anomalous overpressure in the Opalinus Clay (the potential host formation) (Nagra, 2002a).

Site selection and characterisation |
99 |
surround the host formation and the movement of water that takes place through it – this will take place slowly and predominantly vertically, either upwards or downwards in response to the pressure difference across it. When developing such a model, it is important to use natural boundary conditions wherever possible, which are commonly provided by the extent of the more permeable units and which are closely related to those of the geological formations – recharge in areas of outcrop and natural discharge zones provided, e.g., by rivers. Unlike more local models, the regional model also simulates the flow at considerable depth and, because of its scale, it can be used as a basis for longterm assessments in which climatic and geological changes have to be considered. It can also be used to define the boundary conditions for the sub-regional and local models.
4.2.2.3. More local hydrogeological model(s)
A more local model, or possibly models, will also be required to investigate flow on a more local scale. The sub-regional model of Fig. 4.10 has, e.g., an areal extent of approximately 1500 km2 and was designed to obtain knowledge on the directions and rates of groundwater flow on the local scale in the aquifer immediately above the Boom Clay. This was considered important because this aquifer separates the geological barrier of the disposal system (the Boom Clay) from the biosphere, where the radionuclides can come into contact with man. The refinement in the modelling of flow of groundwater within this aquifer was achieved by taking into account a greater level of detail of the hydrographic network, by using a smaller grid, and, thereby, making it possible to take smaller rivers into account and allowing a more detailed differentiation of the hydraulic properties and geometry of the formations.
Geographical extension |
Hydrogeological units -- modelling |
|
|
|||||||||||
|
|
|
|
South-West |
Northeast |
|
|
|
|
|
|
|
||
|
|
|
|
|
|
|
Mol Sands |
-- |
|
Aquifer 1 |
|
|||
|
|
|
|
|
|
|
Kasterlee Sands |
-- |
|
Aquifer 2 |
|
|||
|
|
|
|
|
|
|
Aquitard |
-- |
Aquitard 1 |
|
||||
|
|
|
|
|
|
|
“Lillo/Kasterlee” |
|
|
|
|
|
|
|
|
|
|
|
|
|
|
Diest Sands |
-- |
Aquifer 3 |
|
||||
N |
|
Hreda |
|
|
|
|
Berchem Sands -- Aquifer 4 |
|
||||||
|
|
|
|
|
|
|
||||||||
Bergen op Zoom |
|
|
|
|
|
Voort Sands |
-- |
Aquifer 5 |
|
|||||
|
|
|
|
|
|
|
|
|
|
|
|
|
||
|
|
|
Poppel |
(300 km 2) |
|
|
|
|
|
|
|
|
|
|
|
|
Tumhout |
South-West |
Northeast |
|
|
|
|
|
|
|
|||
|
|
|
|
|
|
|
|
|
|
|
||||
|
|
|
Lummel |
|
|
|
Mol Sands |
|
|
-- |
Aquifer 1 |
|||
Arawerpen |
|
|
|
|
|
|
|
|
|
|
|
|
||
|
Mol |
|
|
|
Kasterlee Sands |
-- |
Aquifer 2 |
|||||||
|
|
|
|
|
|
|||||||||
|
Lier |
|
|
|
|
|
Aquitard |
-- |
Aquitard 1 |
|||||
|
|
|
|
|
|
“Lillo/Kasterlee” |
|
|
|
|
|
|||
|
|
|
|
|
|
|
|
|
|
|
|
|||
|
|
|
|
|
|
|
Diest Sands |
|
|
-- |
Aquifer 3 |
|||
|
|
|
Diest |
|
|
|
Berchem Sands -- Aquifer 4 |
|||||||
|
|
Auruchot |
|
|
|
|
|
|
|
|
|
|
|
|
|
|
Hasselt |
|
|
|
Voort Sands |
|
|
-- |
Aquifer 5 |
||||
|
|
|
|
|
|
|
|
|
|
|
|
|
|
|
Brussel |
Leuven |
|
(1500 km |
2 |
) |
|
|
|
|
|
|
|
|
|
|
|
|
|
|
|
|
|
|
|
|
|
|
|
|
Belgium |
|
|
|
|
|
|
|
|
|
|
|
|
|
|
|
0km |
20km |
|
|
|
|
|
|
|
|
|
|
|
|
|
|
|
|
|
|
|
|
Quaternary |
|
-- |
Aquifer 1 |
|||
Regional |
|
|
Local |
|
|
|
|
Quaternary Clay -- Aquitard 1 |
||||||
Sub-Regional |
|
|
|
|
Brasschaat, Merksplas, |
|||||||||
model |
model |
|
model |
|
|
|
|
Mol and Poederlee -- Aquifer 2 |
||||||
|
|
|
|
|
|
|
|
Lillo-Kasterlee |
-- |
Aquitard 2 |
||||
|
|
|
|
|
|
|
|
Miocene |
|
|
|
-- |
Aquifer 3 |
|
|
|
|
|
|
|
|
|
Boom Clay |
|
-- Aquitard 3 |
||||
|
|
|
|
|
|
|
|
Lower-Rupelian |
-- |
Aquifer 4 |
||||
|
|
|
|
(7000 km 2) |
|
Asse Clay |
|
|
|
-- |
Aquitard 4 |
|||
|
|
|
|
|
Lede-Brussel |
|
-- |
Aquifere 5 |
Fig. 4.10. The three scales of groundwater flow modelling carried out in Belgium as part of SAFIR 2, the regional (lowest), sub-regional (middle) and local (top) models (ONDRAF, 2001a,b).
100 |
T. McEwen |
At the Mol site, a smaller-scale |
model was also developed, covering an area |
of 300 km2 and with boundary conditions determined from the larger-scale models (Fig. 4.10). This model was used as the basis for calculating the migration/dispersion of radionuclides from the repository and needed to have the required degree of detail and be as limited as possible geographically in order to avoid excessive calculation times.
As well as developing an understanding of the groundwater flow system, it is also important, perhaps of greater importance, to develop an understanding of the hydrochemical system – to determine the composition of the groundwaters, to relate these compositions to the mineralogy of the rocks and to determine the chemical evolution of the groundwater system over long periods of time. Geochemical work has two important advantages over purely hydrogeological investigations; one of these is that historical data can be extracted directly from geochemical studies without the uncertainties associated with the long-term extrapolation of groundwater flow models and the second is that a large number of variables can be measured. Most natural systems are likely to provide in excess of 250 constituents which could be measured, each of which will provide some information on one or more pieces of the overall picture, such as the residence time of the groundwater, its chemical characteristics at the time of recharge, the chemical reactions that have taken place, the extent of mixing of different groundwater systems, etc.
The sampling and analysis of groundwater from boreholes forms an essential element in a site investigation and, to ensure the collection of representative samples, the sampling programme must be included in the initial design of the borehole programme. While it is comparatively easy to obtain high-quality groundwater samples in aquifers using sampling systems that preserve the in situ pressure of the groundwater at the sampling depth, it is considerably more difficult to obtain such samples in clays (or any other very low-permeability formations such as an evaporite). In such rocks, the inflow of groundwater into any sampling system is likely to be extremely small and recourse may have to be made to pore fluid extraction from clay samples in the laboratory, by techniques such as squeezing, or by other techniques in the case of evaporites. Extensive work has been carried out over the last decade on the physico-chemical processes in deep clays, as they have become of greater interest for the disposal of radioactive waste, and chemical studies do provide one of the best methods of demonstrating the processes that have taken place in such clays over long periods of time – perhaps as much as several million years (e.g., Nagra, 2002a; ONDRAF, 2001a,b). Similarly, it is possible to demonstrate the slow rates of chemical change that have taken place in evaporites.
Figure 4.11, for example, shows the 2H profile through the Opalinus Clay, which lies between two more permeable formations of the Malm and the Keuper, and a similar profile exists for chloride and other elements. The measured values have been compared with the results of a pure diffusion model and the good fit demonstrates that there is no significant vertical advective flow. The value of such data, which is equivalent to a longterm, natural experiment, is that the results are relevant for long timescales (in this case perhaps as much as many millions of years) and over significant distances (again in this case more than 100 m). Similar results in other clays at depth could also indicate similar times and distances. Data of this type therefore provide convincing evidence of the stability of this type of natural system at depth – something that is of considerable advantage in being able to demonstrate the intrinsic suitability of such an environment for disposal purposes.