
биохимия атеросклероза
.pdf68 Bernardo Trigatti
72.Silver DL: SR-BI and protein–protein interactions in hepatic high density lipoprotein metabolism. Rev Endocr Metab Disord 5: 327–333, 2004.
73.Yesilaltay A, Kocher O, Rigotti A, Krieger M: Regulation of SR-BI-mediated high-density lipoprotein metabolism by the tissue-specific adaptor protein PDZK1. Curr Opin Lipidol 16: 147–152, 2005.
74.Ikemoto M, Arai H, Feng D, Tanaka K, Aoki J, Dohmae N, Takio K, Adachi H, Tsujimoto M, Inoue K: Identification of a PDZ-domain-containing protein that interacts with the scavenger receptor class B type I. Proc Natl Acad Sci USA 97: 6538–6543, 2000.
75.Silver DL: A carboxyl-terminal PDZ-interacting domain of scavenger receptor B, type I is essential for cell surface expression in liver. J Biol Chem 277: 34042–34047, 2002.
76.Silver DL, Wang N, Vogel S: Identification of small PDZK1-associated protein, DD96/MAP17, as a regulator of PDZK1 and plasma high density lipoprotein levels. J Biol Chem 278: 28528–28532, 2003.
77.Kocher O, Yesilaltay A, Cirovic C, Pal R, Rigotti A, Krieger M: Targeted disruption of the PDZK1 gene in mice causes tissue-specific depletion of the high density lipoprotein receptor scavenger receptor class B type I and altered lipoprotein metabolism. J Biol Chem 278: 52820–52825, 2003.
78.Lan D, Silver DL: Fenofibrate induces a novel degradation pathway for scavenger receptor B-I independent of PDZK1. J Biol Chem 280: 23390–23396, 2005.
79.Mardones P, Pilon A, Bouly M, Duran D, Nishimoto T, Arai H, Kozarsky KF, Altayo M, Miquel JF, Luc G, Clavey V, Staels B, Rigotti A: Fibrates down-regu- late hepatic scavenger receptor class B type I protein expression in mice. J Biol Chem 278: 7884–7890, 2003.
80.Burgos PV, Klattenhoff C, de la Fuente E, Rigotti A, Gonzalez A: Cholesterol depletion induces PKA-mediated basolateral-to-apical transcytosis of the scavenger receptor class B type I in MDCK cells. Proc Natl Acad Sci USA 101: 3845–3850, 2004.
81.Kawashima S, Yokoyama M: Dysfunction of endothelial nitric oxide synthase and atherosclerosis. Arterioscler Thromb Vasc Biol 24: 998–1005, 2004.
82.Alp NJ, McAteer MA, Khoo J, Choudhury RP, Channon KM: Increased endothelial tetrahydrobiopterin synthesis by targeted transgenic GTP-cyclohy- drolase I overexpression reduces endothelial dysfunction and atherosclerosis in ApoE-knockout mice. Arterioscler Thromb Vasc Biol 24: 445–450, 2004.
83.Shaul PW: Endothelial nitric oxide synthase, caveolae and the development of atherosclerosis. J Physiol 547: 21–33, 2003.
84.Ozaki M, Kawashima S, Yamashita T, Hirase T, Namiki M, Inoue N, Hirata K, Yasui H, Sakurai H, Yoshida Y, Masada M, Yokoyama M: Overexpression of endothelial nitric oxide synthase accelerates atherosclerotic lesion formation in apoE-deficient mice. J Clin Invest 110: 331–340, 2002.
85.Shi W, Wang X, Shih DM, Laubach VE, Navab M, Lusis AJ: Paradoxical reduction of fatty streak formation in mice lacking endothelial nitric oxide synthase. Circulation 105: 2078–2082, 2002.
86.Trigatti BL: Hepatic high-density lipoprotein receptors: roles in lipoprotein metabolism and potential for therapeutic modulation. Curr Atheroscler Rep 7: 344–350, 2005.
87.Acton SL, Scherer PE, Lodish HF, Krieger M: Expression cloning of SR-BI, a CD36-related class B scavenger receptor. J Biol Chem 269: 21003–21009, 1994.
Chapter 4. Role of the Scavenger Receptor-BI and Atherosclerosis |
69 |
88.Calvo D, Vega MA: Identification, primary structure, and distribution of CLA-1, a novel member of the CD36/LIMPII gene family. J Biol Chem 268: 18929–18935, 1993.
89.Rajapaksha WR, McBride M, Robertson L, O’Shaughnessy PJ: Sequence of the bovine HDL-receptor (SR-BI) cDNA and changes in receptor mRNA expression during granulosa cell luteinization in vivo and in vitro. Mol Cell Endocrinol 134: 59–67, 1997.
90.Shiratsuchi A, Kawasaki Y, Ikemoto M, Arai H, Nakanishi Y: Role of class B scavenger receptor type I in phagocytosis of apoptotic rat spermatogenic cells by sertoli cells. J Biol Chem 274: 5888–5894, 1999.
91.Ritsch A, Tancevski I, Schgoer W, Pfeifhofer C, Gander R, Eller P, Foeger B, Stanzl U, Patsch JR: Molecular characterization of rabbit scavenger receptor class B types I and II: portal to central vein gradient of expression in the liver. J Lipid Res 45: 214–222, 2004.
92.Kim JG, Vallet JL, Nonneman D, Christenson RK: Molecular cloning and endometrial expression of porcine high density lipoprotein receptor SR-BI during the estrous cycle and early pregnancy. Mol Cell Endocrinol 222: 105–112, 2004.
93.Hung AY, Sheng M: PDZ domains: structural modules for protein complex assembly. J Biol Chem 277: 5699–5702, 2002.
Biochemistry of Atherosclerosis edited by S.K. Cheema, Springer, New York, 2006
5
The Role of Scavenger Receptors
in Signaling, Inflammation, and
Atherosclerosis
DAISY SAHOO AND VICTOR A. DROVER
Abstract
The scavenger receptor (SR) family of cell surface, integral membrane proteins has been extensively characterized as mediators of modified lipoprotein binding and internalization. The role of these receptors in atherogenesis is highlighted by their ability to regulate the uptake of cholesterol both from modified low-density lipoproteins in peripheral tissues and cells such as macrophages, as well as from high density lipoprotein in tissues such as the liver and adrenal. However, it is becoming increasingly apparent that like other cell surface receptors, SRs appear to be associated with a plethora of ligand-induced intracellular signaling events that may modulate their impact on atherosclerosis, independent of their effects on cholesterol homeostasis. This chapter will describe the current understanding of these processes for the following SRs: macrophage scavenger receptor-1 (SR-A), CD36, scavenger receptor class B type I (SR-BI), and lectin-like oxLDL receptor-1 (LOX-1).
Keywords: atherosclerosis; CD36; LOX-1; scavenger receptor; signaling; SR-A; SR-BI
Abbreviations: acLDL, acetylated LDL; BAEC, bovine arterial endothelial cells; eNOS, endothelial NO synthase; FH, familial hypercholesterolemia; HCAEC, human coronary artery endothelial cells; HDL, high-density lipoprotein; IL, interleukin; LDL, low-density lipoprotein; LDL-R, LDL receptor; LOX-1, lectin-like oxLDL receptor-1; LPS, lipopolysaccharide; MAPK, mitogen-activated protein kinase; mLDL, modified LDL; NF-κB, nuclear factor-κB; NO, nitric oxide; oxLDL, oxidized LDL; PKC, protein kinase C; PTK, protein tyrosine kinase; ROS, reactive oxygen species; SAA, serum amyloid A; SMC, smooth muscle cell; SR, scavenger receptor; SR-A, macrophage scavenger receptor-1; SR-BI, scavenger receptor class B type I; TLR, toll-like receptor; TNF, tumor necrosis factor; uPA, urokinase-type plasminogen activator; WT, wild type
Introduction
Atherosclerosis is a chronic inflammatory state characterized by the formation of arterial plaques—lesions resulting from the accumulation of choles- terol-rich macrophages in the intimal space of the vessel wall. Left untreated,
70
Chapter 5. The Role of SRs in Signaling, Inflammation, and Atherosclerosis |
71 |
atherosclerosis can lead to hypertension, myocardial infarction, stroke, and death. Scavenger receptors (SRs) are membrane-bound proteins and play a critical role in the pathology of atherosclerosis via their ability to bind lipoproteins and internalize their lipid cargo. In addition to cholesterol transport, SRs can also mediate intracellular signaling and inflammatory responses. Given the increasingly important role of these processes in the development and progression of atherosclerosis, characterizing the specific signaling functions of SRs is crucial to a thorough understanding of disease progression. We begin with a brief description of the pathology of atherosclerosis. We will then describe the potential roles of SRs in regulating intracellular signaling pathways involved in atherogenesis.
Atherosclerosis: A Chronic Inflammatory State
A hallmark feature of atherosclerosis is the presence of white, lipid-laden “fatty streaks” in the arterial wall. Fatty streaks or plaques are thickenings of the intima, the innermost layer of the artery wall, and consist primarily of neutral lipids (such as cholesteryl esters), apoptotic cellular debris, connective tissue, and inflammatory/immune cells including macrophages and T cells. These lesions are commonly found in young persons and, with age, may disappear or develop into advanced plaques. A developing lesion can grow large enough to protrude into the arterial lumen, reduce blood flow, and thus cause hypertension. If the plaque ruptures, the resultant thrombosis can completely block the artery leading to myocardial infarction, stroke, and/or death.
Once thought to be a simple disorder of lipid metabolism, we now recognize that atherosclerosis is a complex process whereby lipoproteins interact with inflammatory cells in the intima to produce foam cells rich in cholesteryl esters. The following sections will describe these interactions in four stages: lesion initiation, monocyte infiltration and differentiation, lesion progression, and plaque rupture.
Lesion Initiation
Lesion formation in the intimal space is thought to occur at sites of endothelial perturbation such as vessel injury, turbulent blood flow, and lipoprotein infiltration [1]. While controversy exists as to the whether arterial inflammation precedes the lipid accumulation or vice versa [2]—a matter which is further complicated by the fact that hypercholesterolemia is itself associated with inflammation [3]—it is clear that a close relationship exists between these two processes during lesion formation.
Since lipoprotein penetration is directly related to the low-density lipoprotein (LDL)-associated cholesterol concentration in the plasma, LDL levels are a major indicator for the development of atherosclerosis [4]. When LDL penetration occurs at sites of hemodynamic strain (such as vessel bifurcation),
72 Daisy Sahoo and Victor A. Drover
the inflammatory process begins and LDL can be oxidatively or enzymatically modified (reviewed in [5]). LDL modification results in the release of oxidized phospholipids and lysophospholipids in the intimal space. These bioactive lipids activate the endothelial cells lining the arterial wall, resulting in the expression of adhesion molecules, chemotactic proteins, and cytokines (reviewed in [6]).
Monocyte Infiltration and Differentiation
Monocytes adhere to the endothelium via receptors for adhesion molecules and chemotactic proteins and readily enter the intima [7]. Cytokines present in the intimal space promote (i) monocyte differentiation into macrophages, (ii) SR expression, and (iii) the internalization of modified LDL (mLDL) [8, 9]. Like the uptake of native LDL through the classical LDL receptor (LDL-R) pathway [10], the cholesterol derived via the SR pathway exerts feedback repression on additional cholesterol synthesis and uptake via the LDL-R. However, the expression of SRs is not downregulated by excess cellular cholesterol. Rather, SR expression is increased by oxidized LDL (oxLDL) [11] and macrophages in the intimal space accumulate massive amounts of cholesterol/cholesteryl esters as a result. These cholesterolloaded macrophages are called foam cells and are the main constituents of fatty streaks.
Lesion Progression
The inflammatory environment of the fatty streak/early plaque activates T cells to produce a host of proand anti-inflammatory cytokines, which potentiate atherosclerosis [12, 13]. The transition from a fatty streak to a complex plaque depends upon a vicious inflammatory cycle of T cell and macrophage activation. This in turn causes smooth muscle cell (SMC) migration, additional foam cell formation, and the production of extracellular matrix proteins which form a fibrous cap over the plaque protruding into the lumen of the artery. Cellular lipid accumulation in the increasingly inflammatory environment of the intimal space triggers apoptosis and necrosis of macrophageand SMC-derived foam cells, producing a core of acellular debris. As a result, advanced lesions contain a number of cell types not abundant in early fatty steaks including SMCs, mast cells, and T cells. In addition, a late-stage plaque has a core of insoluble lipid and apoptotic cellular debris covered by a fibrous cap.
Plaque Rupture
Although arterial narrowing may cause some symptoms of atherosclerosis (such as hypertension), myocardial infarction and stroke likely result from plaque rupture (reviewed in [14]). Once the thrombotic material below the
Chapter 5. The Role of SRs in Signaling, Inflammation, and Atherosclerosis |
73 |
fibrous cap (i.e., plaque lipids and tissue factor) come into contact with the blood, the coagulation cascade begins. Subsequent platelet adhesion and thrombus formation eventually occludes blood flow. Advanced plaques may also develop through a number of smaller plaque ruptures and thrombotic events, which successively narrow the artery prior to a major ischemic rupture.
The stability of an atherosclerotic lesion is very much dependent on the integrity of the fibrous cap [14]. Thus, lesions with thin fibrous caps or large necrotic cores are particularly susceptible to rupture. The “shoulder region” where the edges of the fibrous cap meet the arterial wall is a frequent site of plaque rupture and a relative accumulation of foam cells in this area is commonly observed. The secretion of matrix metalloproteinases by foam cells close to the shoulder region may result in local degradation of the fibrous cap, thus leading to plaque rupture.
Scavenger Receptor Classification, Functions, and
Regulation of Inflammation/Signaling
The identification of SRs has its roots in the characterization of the LDL-R pathway by Goldstein and Brown (reviewed in [10]). LDL, the most abundant source of plasma cholesterol in humans, is internalized and degraded via the cell surface LDL-R. Cholesterol acquired by this pathway exerts feedback repression on de novo cholesterol synthesis as well as additional LDL- R-mediated cholesterol uptake. Mutations in the LDL-R result in familial hypercholesterolemia (FH), a disease that presents with abnormally high levels of cholesterol in the plasma [15]. Additional symptoms include delayed clearance of LDL from the plasma and heart attacks in early childhood. Importantly, cholesterol still accumulates in macrophages, kidney, skin, and other tissues in FH patients, suggesting an alternate “scavenging” cholesterol transport pathway.
One hypothesis put forth from the work described above was that the delayed clearance of LDL in FH patients might result in a chemical modification of the lipoprotein, thus making it available to the scavenger pathway in vivo. Based on this premise, acetylated LDL (acLDL) was used to identify a high-affinity binding site in liver and macrophages that did not recognize native LDL [16]. The uptake of acLDL via this alternate pathway caused massive cellular cholesterol deposition due to a lack of feedback regulation. AcLDL binding was then used to isolate the first SR [17] and the partial protein sequence was used to clone the cDNA [17, 18].
After more than a decade of research, mLDL binding remains the unifying trait of the SR family, which is now composed of a diverse group of integral membrane proteins divided into at least eight classes (reviewed in [19]). In addition to binding mLDL and/or other lipoproteins, a wide range of additional ligands exists for this protein family such as fatty acids, oxidized
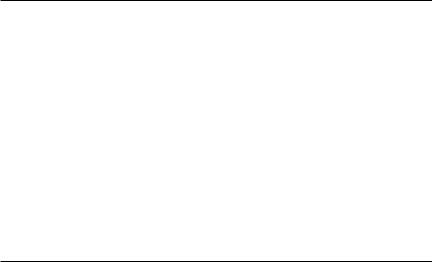
74 Daisy Sahoo and Victor A. Drover
phospholipids, apoptotic cells, and bacterial lipopolysaccharides (LPS; see Table 5.1). Together, the SRs exert significant effects on cholesterol and fat metabolism as well as innate immune function. While these proteins have been studied primarily in regard to their ability to mediate ligand clearance, it is now becoming clear that intracellular signaling pathways initiated by these ligands extend the functionality of SRs into many metabolic processes. However, due to their recent classification or identification, little or no information is available for some receptors in regard to signaling, inflammation, or atherosclerosis. Thus, this chapter will focus on some of the most well-described SRs—macrophage scavenger receptor-1 (SR-A), CD36, scavenger receptor class B type I (SR-BI), and lectin-like oxLDL receptor-1 (LOX-1)—which may be involved in the development and progression of atherosclerosis. The impact of intracellular signaling on the expression of SRs will not be discussed so that we can examine the converse: how SR expression regulates inflammation and related signaling pathways. In addition, much of the early characterization of the SR family utilized “specific” ligands to make conclusions about a particular SR. We now recognize that many SR ligands (e.g., oxLDL) can engage multiple receptors. Therefore, we will restrict our discussion to experimental techniques, such as targeted gene disruption or mutagenesis, which clearly identify the appropriate receptor.
TABLE 5.1. Properties of the scavenger receptor family.
|
|
|
|
|
Ligands |
|
|
|
|
|
Primary |
Modified |
Apoptotic |
Bacterial/ |
Oxidized |
Class |
Members |
Topology |
membrane |
LDLa |
cells |
LPS |
lipids |
A |
SR-AI/II, |
Type II |
PM |
+ |
+ |
+ |
NR |
|
MARCO, |
|
|
|
|
|
|
|
SRCL |
|
|
+ |
+ |
+ |
+ |
B |
CD36, SR-B, |
Type III |
PM |
||||
|
LIMP-II |
|
|
+ |
|
+ |
|
C |
dSR-CI |
Type I |
PM |
NR |
NR |
||
D |
CD68, LAMP-1, Type I |
Lysosome |
+ |
NR |
NR |
NR |
|
|
-2, -3 |
|
|
+ |
+ |
+ |
|
E |
LOX-1 |
Type II |
PM |
NR |
|||
F |
SREC-I, |
Type I |
PM |
+ |
+ |
NR |
NR |
|
SREC-II |
|
|
+ |
|
+ |
|
G |
SR-PSOX/ |
Type I |
PM |
NR |
NR |
||
|
CXCL16 |
|
|
+ |
|
+ |
|
H |
FEEL-1, |
Type I |
PM/ |
NR |
NR |
||
|
FEEL-2 |
|
endosome |
|
|
|
|
+, ligand binding reported.
NR, ligand binding not reported. aIncludes oxidized or acetylated LDL.
Chapter 5. The Role of SRs in Signaling, Inflammation, and Atherosclerosis |
75 |
Macrophage Scavenger Receptor-1 (SR-A)
SR-A was the first scavenger receptor to be identified and cloned [17, 18]. It is a homotrimeric, type II integral membrane protein (single transmembrane domain with a cytoplasmic N-terminus) expressed in macrophages, endothelial cells, and smooth muscle tissue. It can bind mLDL/oxLDL and may account for as much as 30% of oxLDL uptake by macrophages [20]. SR-A also binds bacterial components and is an important factor in controlling endotoxemia [21].
The contribution of SR-A to the development of atherosclerosis is currently a controversial subject despite the in vitro evidence that mLDL can engage and be internalized by SR-A [20, 22]. Babaev et al. [23] demonstrated that SR-A−/− C57BL/6 mice on a butterfat diet had smaller lesions in the proximal aorta without a significant change in plasma cholesterol levels [23]. In addition, lethally irradiated C57BL/6 or LDL-R−/− mice reconstituted with SR-A−/− fetal liver cells were protected from lesion formation compared to mice reconstituted with SR-A+/+ control cells. These data corroborate an earlier finding in apoE−/− mice [17] and suggest that SR-A expression is atherogenic due to its ability to mediate the uptake of mLDL. In contrast, Herijgers et al. [24] found that SR-A had no effect on lesion area in LDL-R−/− mice fed a “Western-type” diet and transplanted with bone marrow from mice overexpressing SR-A [24]. The observation that macrophage-specific overexpression of SR-A decreases atherosclerosis in LDL-R−/− mice fed a diet enriched in fat and cholesterol also contradicts the notion that SR-A is atherogenic [25], as does a recent report using apoE−/− SR-A−/− double-null mice [22]. Thus, it seems likely that SR-A can mediate both proand antiatherogenic processes in vivo and the overall susceptibility to lesion formation depends on diet and genetic background of the animal model.
Although the mechanisms which underlie the ability of SR-A to promote or inhibit lesion formation require further study, a small body of work suggests that SR-A may achieve some of its functions via intracellular signaling processes. Sequence analysis of SR-A revealed the presence of three conserved phosphorylation sites in the cytoplasmic domains [26], giving the first indication that SR-A was part of a signaling pathway. In addition, phosphorylation at serine 21 or 49 of mouse SR-A appears to modulate the efficiency of mLDL internalization and metabolism in COS- 7 cells [27]. Mice, which lack c-Jun N-terminal kinase 2, display reduced foam cell formation, reduced uptake of mLDL by macrophages, and reduced SR-A phosphorylation [28]. However, SR-A is not only a target of intracellular signaling. In rat peritoneal macrophages, acLDL (a signature SR-A ligand) stimulated tumor necrosis factor (TNF)-α secretion and increased intracellular calcium levels [29]. The authors showed that TNF-α production could be blocked by inhibitors to heterotrimeric G-proteins,
76 Daisy Sahoo and Victor A. Drover
protein kinase C (PKC), and protein tyrosine kinases (PTK). Inhibiting heterotrimeric G-proteins with pertussis toxin also appears to reduce acLDL uptake, thus indicating a positive feedback pathway between G-proteins and SR-A [30]. Such a pathway may partially explain the continued uptake of mLDL via the SR pathway despite high levels of intracellular cholesterol.
Perhaps the best evidence for a direct link between SR-A and intracellular signaling pathways comes from the plasminogen activator field. Urokinase-type plasminogen activator (uPA) is a protease expressed by macrophages in the arterial wall; in human arterial lesions, uPA levels are elevated and correlate directly with the severity of atherosclerotic lesion formation [31]. In THP-1 macrophages, acLDL increases the steady-state levels of uPA in a PTKand PKC-dependent fashion [32]. AcLDL also stimulates a dramatic increase in cellular protein tyrosine phosphorylation in these cells as well as in Bowes human melanoma cells transfected with SR-A. In contrast, the parental Bowes cells, which do not express SR-A, showed limited protein tyrosine phosphorylation upon acLDL incubation [32]. The requirement of SR-A expression for protein tyrosine phosphorylation and elevated uPA levels strongly implicate SR-A in intracellular signaling pathways. Interestingly, the exact role of uPA in lesion formation is not clear due to discrepancies between various studies similar to the discrepancies reported for SR-A (discussed earlier). One might speculate that the atherogenic potential of SR-A is related to its ability to induce or repress uPA levels. However, prospective studies are required to confirm this notion.
SR-A also appears to mediate the activation of the Src-family of PTKs. Miki et al. [33] have reported that acLDL rapidly induces the phosphorylation and activation of Lyn in THP-1 macrophages. Under identical conditions, Lyn was coimmunoprecipitated with an α-SR-A antibody. The same group more recently reported that Lyn−/− mice have reduced atherosclerosis despite elevated plasma cholesterol [34], but did not test SR-A function directly. It is imperative to determine whether the atheroprotection observed in this strain is due to SR-A dysfunction, and whether Lyn-mediated signaling pathways are altered in SR-A−/− mice.
Finally, SR-A plays an important but apparently indirect role in mediating inflammation induced by bacterial glycolipids such as endotoxin or LPS. SR-A−/− mice are more susceptible to LPS-induced death compared to wildtype (WT) mice [21]. This phenotype appears to be due to an elevated inflammatory response as serum TNF-α and interleukin (IL)-6 levels are higher in the absence of SR-A and survivability of SR-A−/− mice injected with LPS is restored when pretreated with a TNF-α blocking antibody. The mechanism for the sensitivity to endotoxic shock is unclear due to conflicting reports on the half-life of serum LPS in WT and SR-A−/− mice [35, 36]. Thus, it is not known what role SR-A has, if any, on modulating the downstream signaling events following LPS activation.
Chapter 5. The Role of SRs in Signaling, Inflammation, and Atherosclerosis |
77 |
CD36
CD36, also known as FAT, GPIV, and SCARB3, is a type III (multiple transmembrane domains) membrane glycoprotein expressed in numerous tissues (adipose, heart, muscle, intestine) and cell types (monocytes/macrophages, microvascular endothelium, dendritic cells, retinal epithelia, SMCs). It is predicted to have a “hairpin” topology (a large extracellular loop and two transmembrane domains with both the C- and N-termini projecting into the cytoplasm) and has been implicated in numerous cellular functions including atherosclerosis (reviewed in [37]). This broad range of functions is reflected by the numerous ligands for this receptor, which include thrombospondin, anionic phospholipids, β-amyloid, long-chain fatty acids, native lipoproteins, and oxLDL.
In an atherogenic background, CD36−/− mice appear to be protected from plaque formation and atherosclerosis [38] despite only subtle changes in plasma cholesterol [39]. Bone marrow transplantation studies have also shown that the proatherogenic effects of CD36 are dependent upon bone marrow-derived cells (i.e., monocytes/macrophages) [40]. In vitro experiments demonstrating that CD36 is the major oxLDL receptor in macrophages are consistent with an atherogenic role of CD36 [20, 41, 42]. However, a recent report has called into question the relevance of CD36 in atherogenesis [22] and will likely spark renewed interest in this area to resolve the controversy.
Although the mechanism of atheroprotection in CD36−/− mice likely involves reduced oxLDL/cholesterol uptake by macrophages, a growing body of evidence suggests that CD36 may also play a role in regulating inflammatory responses via the transcription factor nuclear factor-κB (NF-κB; see Fig. 5.1 for a description of the NF-κB pathway). Indirect evidence for this link comes from the observation that oxLDL can modulate NF-κB activity (reviewed in [43]). The first direct evidence was reported by Lipsky et al. [44] using Chinese hamster ovary cells transfected with an expression vector encoding CD36. Preincubating the cells with oxLDL increased the amount of activated NF-κB following exposure to TNF-α. However, this was not observed when cells were transfected with a vector encoding a mutant CD36 lacking the last (3′) six codons. Janabi et al. [45] further showed that oxLDL induction of NF-κB activation was defective in monocyte-derived macrophages from CD36-deficient human patients. Thus, it is reasonable to conclude that an intracellular protein interacting with the C-terminal cytoplasmic tail of CD36 mediates the effects of oxLDL on NF-κB. In line with this, Bull et al. [46] reported a physical interaction between CD36 and Srcrelated PTKs Fyn, Lyn, and Yes in human dermal microvascular endothelial cells. Moore et al. [47] independently verified these coimmunoprecipitation studies between CD36 and Lyn in elicited murine peritoneal macrophages treated with a nonlipid CD36 ligand and further showed that this interaction was required for subsequent, ligand-induced mitogen-activated protein kinase (MAPK) activation [47]. In COS-7 cells, an interaction between CD36